Fig. 3.1
Dual layer CT technology. Dual-layer CT detectors separate-ray photons into two distinct energy spectra. The first (or top) layer encountered by photons absorbs the low-energy spectrum and the bottom detector layer absorbs the remaining higher energy photons Note: The separation of the detection layers is for illustrative purposes only. In reality, the detector layers are in physical contact, one with the other
Principles of Image Reconstruction
The X-ray attenuation of a material can be expressed as a linear combination of its Compton scatter and photoelectric coefficients and by extension the attenuation coefficients at low-energy and high-energy can be expressed as the attenuation contributions from a predefined pair of basis materials [1]. The photoelectric effect is dominant at lower photon energies and relatively high for high atomic number (Z) materials exhibiting an approximately Z 4 relationship. Compton scattering on the other hand is dominant at higher photon energies and is proportional to Z. This effect is generally independent of the electron density of the material. From a given pair of data at two different energies, a new set of images can be generated that have been decomposed into components of a basis pair of materials or, alternatively, individual Compton scatter and photoelectric components can be created [2].
With the dual layer detector, projection space decomposition is used to first solve the individual basis components (Photoelectric and Compton basis pair) and then reconstruct images of the bases. Projection based decomposition requires spatial and temporal alignment, which is achieved with the dual layer detector. A major advantage of projection space decomposition, compared to image space decomposition, is that the material make up is known at each sample which permits more accurate beam hardening correction [3]. This enables the reconstruction of virtual monochromatic images with accurate beam hardening correction. It also enables reconstruction of specific material basis pairs (e.g. iodine and water), material specific and effective atomic number images (Fig. 3.2).
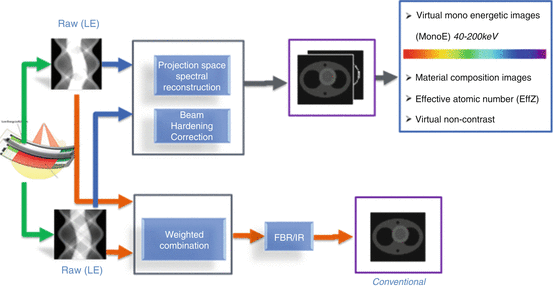
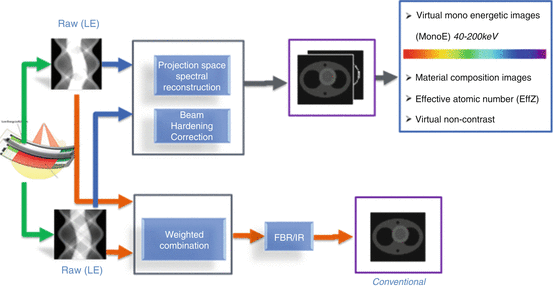
Fig. 3.2
The image reconstruction process for generating spectral images. The low- and high-energy data streams are decomposed into photoelectric and Compton scatter basis pair. This enables the synthesis of virtual monochromatic images, effective atomic number, and material specific images
Types of Images
Dual-layer detector CT can be used to generate several types of images for various clinical applications. Image types include conventional polychromatic, virtual monochromatic, material composition (e.g., iodine only, and virtual non contrast) and effective atomic number based CT images.
Conventional CT Images
Dual layer detectors simultaneously acquire high and low- energy data. The data are combined to create conventional (i.e., 120 kVp) data and used to generate true conventional images. Conventional data can be reconstructed using standard techniques such as filtered back-projection or iterative reconstruction and images are equivalent to a conventional CT image obtained from a conventional scanner (e.g., Philips Brilliance iCT) with poly-energetic X-ray spectra (Fig. 3.3). This is typically the image type used for interpretation on a routine basis. Additionally, other types of images can be generated from a dual-layer detector scanner on demand. From the combined low and high energy data, photoelectric and Compton basis pair raw data is generated, which then undergoes FBP, beam hardening correction and de-noising to generate photoelectric and Compton images respectively. Depending on how these are post-processed, additional image sets are generated.
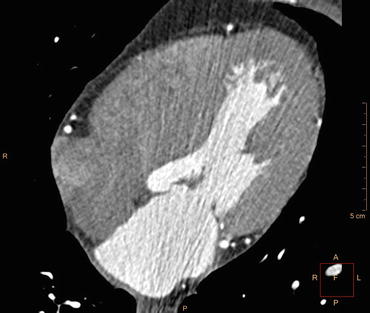
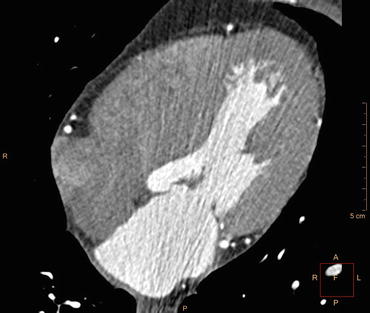
Fig. 3.3
Conventional image of a normal heart obtained from a dual-layer detector scanner. True conventional images are obtained from the conventional data stream which is a combination of the high- and low- energy data acquired. The conventional data steam can be reconstructed using standard reconstruction techniques such as filtered back-projection or iterative reconstruction and images are equivalent to a conventional 120 kVp polychromatic image
Virtual Monochromatic Images
From the basis pair of images, virtual monochromatic images can be generated by the process of linear combination, as per the approach described by Alvarez and Macovski [2]. This model is accurate in the energy range between 30 and 200 keV. Virtual monochromatic images enable the synthesis of images with attenuation properties that are similar to that of an image created with a true mono-energetic beam. These images can be generated from 40 to 200 keV with the dual-layer detector CT scanner (Fig. 3.4).
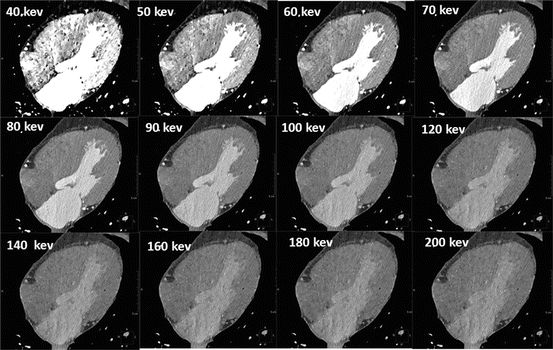
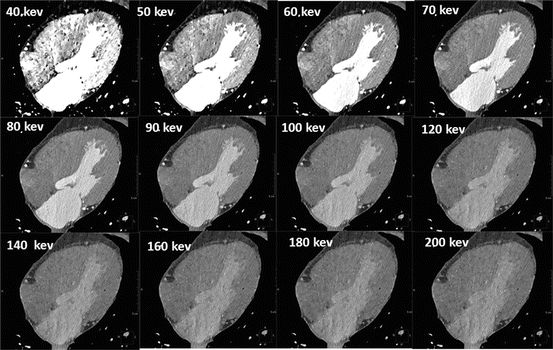
Fig. 3.4
Virtual monochromatic images of a normal heart at different energy levels. From the spectral raw data, virtual monochromatic images can be generated from 40 to 200 keV. At low keV, contrast is high, but noise is also high. At higher monoenergetic levels, contrast and noise are lower
Virtual monochromatic imaging exploits the energy dependence of attenuation properties of materials. For example, generation of images at energies near the k-edge of injected contrast media can be used to boost vascular enhancement. Iodine has a k-edge at about 33 keV so lower energies produce higher levels of attenuation from iodine. Similarly, the k-edge of gold is approximately 80 keV and to enhance the contrast of gold mixtures or compounds, energies of greater than 80 keV can be selected. Virtual monochromatic imaging can also be used to target portions of the energy spectrum for greater artifact reduction. Metal and beam hardening artifacts, for example, are minimized at higher energies.
Material Composition Images
Through the material decomposition process, several types of material composition images can be generated. For example, an iodine-only image represents the presence and concentration of iodine in each voxel; this image type provides a map of iodine flow (Fig. 3.5). Iodine only images are useful for evaluating perfusion of myocardium, lungs or tumors.
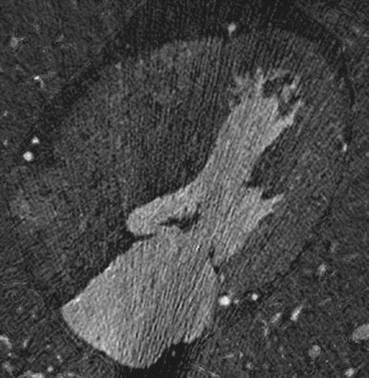
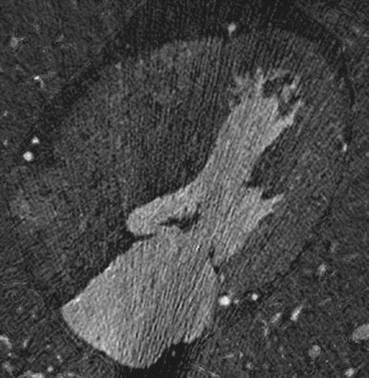
Fig. 3.5
Iodine-only image of a normal heart. Material decomposition was used to generate the image which represents the presence and concentration of iodine in each voxel, thus providing a map of perfusion and iodine flow
In a similar way, all iodine can be removed from an image, thus generating a virtual non-contrast image. The advantage of virtually generated non-contrast images from contrast-enhanced images is the elimination of data acquisition during the non-contrast phase of a multi-phase study, thus saving radiation dose. Additionally, there may be situations where non-contrast data were not acquired but a non-contrast image would aid in the characterization of a lesion (e.g., adrenal lesion, renal lesions).
Effective Atomic Number Based Images
From a combination of photoelectric and Compton images, images based on effective atomic number can be generated from dual-layer data (Fig. 3.6). Discrimination of tissues on the basis of effective atomic number allows a level of discrimination beyond that provided by attenuation alone. For example, distinguishing between iodinated contrast and calcification in a vessel can be challenging because both present with the same Hounsfield unit value [4]. However, an effective atomic number image depicts the material make-up of each voxel and allows differentiation between calcium and iodine. Similarly, an effective atomic number image may be useful in the characterization of non-calcified plaques with similar attenuation properties and Hounsfield Unit values.
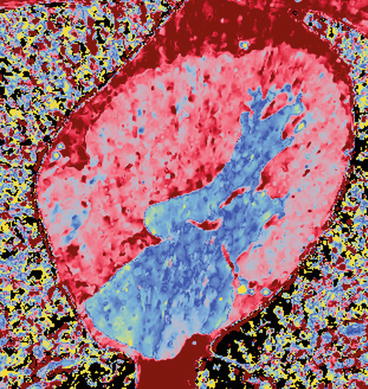
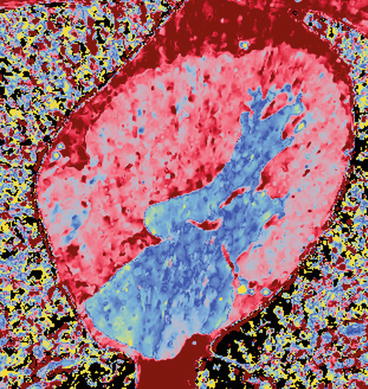
Fig. 3.6
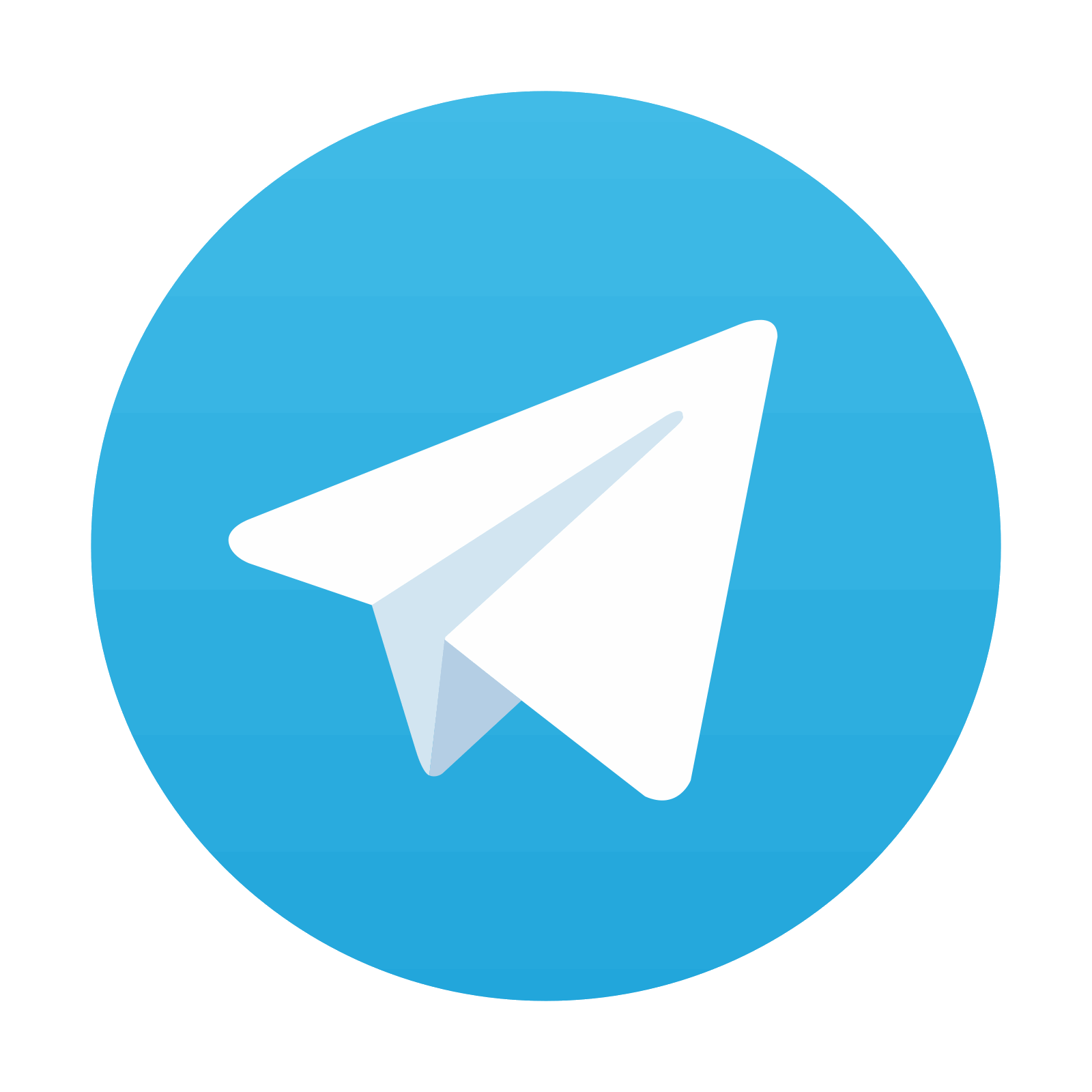
Effective atomic number weighted image of a normal heart. Using material decomposition, an image based on the effective atomic number of tissues was generated. A specific color was assigned to each tissue based on its effective atomic number
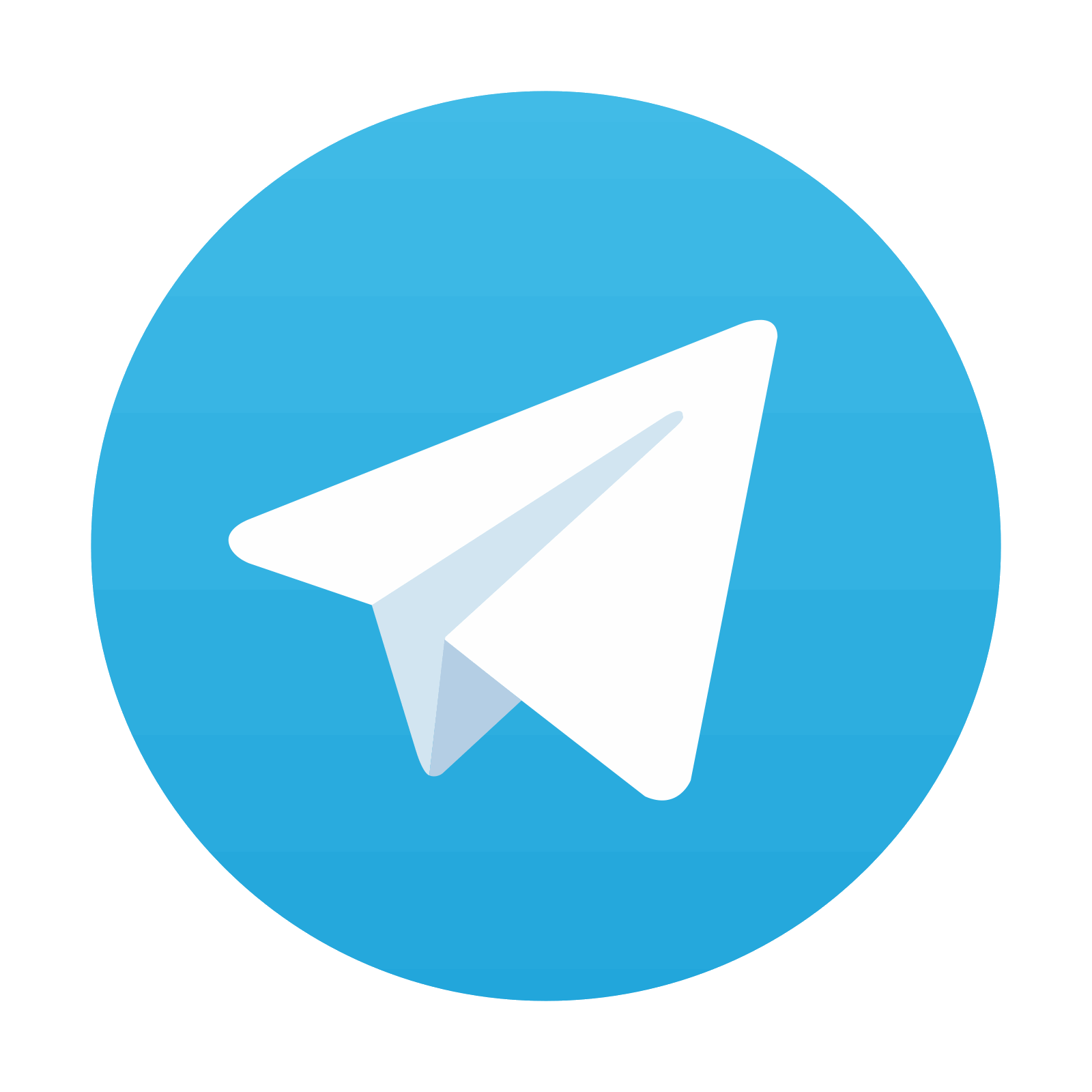
Stay updated, free articles. Join our Telegram channel
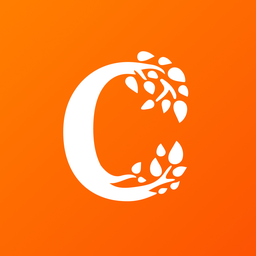
Full access? Get Clinical Tree
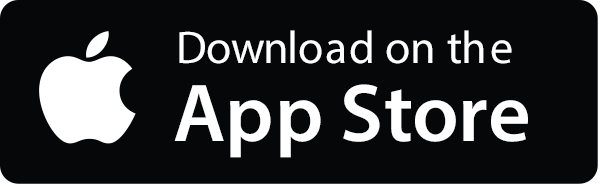
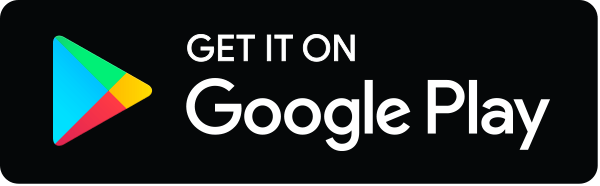
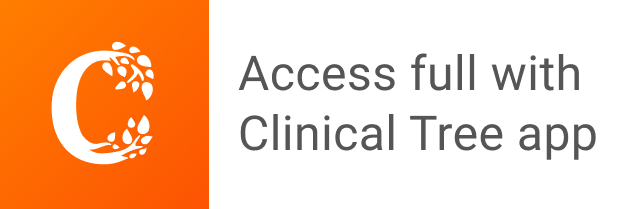