Many disease processes alter the local molecular environment of the myocardium, and consequently the longitudinal (T1) and transverse (T2) relaxation times can change. While such changes may be observed directly as changes in image contrast, the tissue processes may be global and diffuse, hampering reliable detection of the disease. Quantitative methods for characterizing myocardial tissue based on parametric mapping of T1 and T2 have been proposed as an objective way of detecting and quantifying both focal and global changes in tissue. In combination with extracellular contrast agent injection, T1 mapping can also provide an estimate of the extracellular volume (ECV) fraction.
Native T1 mapping, as well as ECV mapping, is currently being explored as a diagnostic tool for a wide range of cardiomyopathies, and native T1 changes are detectable in both acute and chronic myocardial infarction (MI) and may be used to characterize the edematous area at risk. Elevated native T1 has also been reported in a number of diseases with cardiac involvement (e.g., myocarditis, amyloidosis, lupus, and system capillary leakage syndrome ), and decreases in native T1 have been associated with Anderson-Fabry disease and high iron content. Native T2 mapping is used to detect edema in acute MI and myocarditis and to identify the area at risk in acute MI. Application of tissue characterization using parametric mapping for detection of disease is discussed elsewhere in this book.
This chapter describes state-of-the-art methods for measuring T1, T2, and ECV in the myocardium. Because the purpose of such techniques is a quantitative (objective) evaluation of biomarkers and disease, the discussion of the available techniques is framed in terms of accuracy, precision, and general reliability. In parametric mapping it is important to understand errors in quantification and other artifact mechanisms, which may be less familiar than conventional cardiovascular magnetic resonance (CMR) artifact mechanisms. Method imperfections may manifest themselves as subtle changes in measured tissue parameters, and artifactual changes may well be correlated with specific patient groups—that is, they are potential confounders. In particular, the estimated parameter values such as T1 may be affected by other variables such as myocardial wall thickness, T2, protocol settings, or scanner adjustments. Although this is not new to magnetic resonance imaging (MRI), where T1-weighted contrast may have some T2 contrast, these confounding effects may influence the interpretation of parametric maps. Normal values for parameters may depend on the field strength and the specific measurement technique or imaging protocol. Therefore well-controlled and optimized protocols are key to reproducibility, which is particularly important in applications aimed at the detection of subtle fibrosis and preclinical disease, and normal values need to be established for specific protocols. Limited spatial resolution will lead to errors caused by partial volume effects, particularly between myocardium and adjacent blood pool or fat tissue. Despite limitations, parametric mapping is a powerful tool in the assessment of diffuse myocardial disease.
T1 and Extracellular Volume Mapping
Changes in both native T1 and T1 following the administration of gadolinium (Gd) contrast agents are considered important biomarkers, and multiple methods have been suggested for quantifying myocardial T1 in vivo. In general, methods for measuring myocardial T1 consist of three components: (1) a perturbation of the longitudinal magnetization (i.e., an inversion or saturation), (2) an experiment to sample the relaxation curve as the longitudinal magnetization returns to its original level, and (3) a model used to fit the sampled curve and extract the myocardial T1. This chapter focuses on the technical aspects of key methods and imaging protocols and describes their limitations and the factors that influence their accuracy, precision, and overall reproducibility. The accuracy and precision of these measurements affect the detection and quantification of abnormal myocardial tissue.
Late gadolinium enhancement (LGE) is currently the primary tool for tissue characterization in CMR because it provides excellent depiction of MI and focal scar and has become an accepted standard for assessing myocardial viability. LGE is also useful for detecting and characterizing fibrosis that is “patchy” in appearance, for example, as seen in nonischemic cardiomyopathies such as hypertrophic cardiomyopathy (HCM). Diffuse myocardial fibrosis is, however, more difficult to distinguish using LGE, since the myocardial signal intensity may be nearly isointense and may be globally “nulled,” thus appearing to be normal tissue. Alternatively, quantitative measurement of myocardial T1 following the administration of an extracellular Gd contrast agent has been shown to be sensitive to increased ECV associated with diffuse myocardial fibrosis. However, a single postcontrast T1 measurement has limitations because of a variety of confounding factors such as Gd clearance rate, time of measurement, injected dose, body composition, and hematocrit. These factors cause a significant variation in postcontrast T1, making it difficult to distinguish diseased and normal tissue based on absolute T1 values alone. Precontrast T1 varies with water content and may be elevated in cases of diffuse myocardial fibrosis. Precontrast T1 also varies significantly with field strength. Direct measurement of ECV was initially developed for quantifying the myocardial extracellular fractional distribution volume and has been proposed as a means for detection and quantification of diffuse myocardial fibrosis. This approach is based on the change in T1 following administration of an extracellular contrast agent and circumvents the limitation of a single postcontrast T1 measurement in detecting a global change in T1. Myocardial ECV is measured as the percent of tissue composed of extracellular space, which is a physiologically intuitive unit of measurement and is independent of field strength. ECV has been shown to correlate with collagen volume fraction in some diseases.
Brief History of Methods for T1 Mapping in the Heart
Methods for measuring myocardial T1 were initially based on region of interest (ROI) analysis rather than pixelwise parametric maps. Inversion recovery (IR) images at different inversion times were acquired with multiple breath-holds or IR cine protocols were used as a means of acquiring data in a single breath-hold. These early methods were ROI-based schemes and were not suitable for pixelwise mapping. Pixelwise T1 mapping was introduced with the modified Look-Locker inversion recovery (MOLLI) imaging strategy, which propelled the use of T1 mapping in CMR and inspired many new methods. MOLLI is widely used today with some protocol optimization and other adaptations. A shortened breath-hold adaptation with conditional curve fitting (ShMOLLI) was proposed as a means of mitigating heart rate dependence as well as shortening the breath-hold. Further protocol optimization has been aimed at shortening the breath-hold and optimizing precision. Motion correction was developed to mitigate respiratory motion for subjects with poor breath-holding, and phase-sensitive IR reconstruction with motion correction further improved image quality. A number of publications analyzed the accuracy of T1 measurements, leading to a better understanding of the influence of various protocol parameters on T1 measurement errors. Saturation recovery (SR) methods developed initially for T1 measurements during first-pass contrast-enhanced perfusion (short acquisition period-T1 [SAP-T1]) have been recently adapted for T1 mapping using SR single-shot acquisition (SASHA), with steady-state free precession (SSFP) readout as a means of mitigating the T1 underestimation in MOLLI and reducing the influence of confounding factors such as T2, magnetization transfer (MT), heart rate, and off-resonance. Even more recently, hybrid schemes have been proposed that incorporate both inversion and SR methods (saturation pulse prepared heart rate independent inversion-recovery [SAPPHIRE]). ECV measurements were initially introduced using ROI-based measurement, and pixelwise ECV mapping was later introduced. Improvements to T1 and ECV mapping are continuously introduced, such as navigated methods for higher-resolution three-dimensional (3D) or two-dimensional (2D) multislice.
This chapter focuses on the basic concepts behind the widely used MOLLI and SASHA acquisition strategies for T1 mapping, followed by a review of factors influencing accuracy and precision. Discussion includes a description of other limitations and a summary of the pros and cons of various protocols.
T1-Mapping Methods
The currently used protocols for T1 mapping in the heart ( Table 2.1 ) are based on IR or SR. Images are acquired at multiple time points on the recovery curve, and pixelwise curve fitting is performed to estimate the relaxation time parameter to produce a pixel map of T1. Images are generally acquired at the same cardiac phase and respiratory position to eliminate tissue motion. Although initial implementation involved multiple breath-holds, current methods generally use single breath-hold protocols with single-shot 2D imaging. To achieve higher spatial resolution and/or 3D imaging, segmentation may be required.
Inversion recovery (IR) | Multiple breath-hold FLASH |
MOLLI | |
ShMOLLI | |
Modified MOLLI protocols | |
Saturation recovery (SR) | SAP-T1 |
SASHA | |
SASHA VFA | |
SMART1 | |
Combined IR/SR | SAPPHIRE |
MOLLI, the original scheme, was proposed by Messroghli et al. and is illustrated in Fig. 2.1 . For each inversion, the MOLLI method samples the IR curve at multiple inversion times using single-shot imaging spaced at heartbeat intervals. Multiple inversions are used with different trigger delays to acquire measurements at different inversion times to sample the IR curve more finely. Recovery periods are needed between the inversions to ensure that samples from the different inversions are from the same recovery curve; that is, each inversion starts at the same initial condition. The T1-map precision is related to the number and position of samples along the IR curve, and accuracy of the signal model is also affected by the sampling strategy due to the influence of the readout on the apparent recovery.
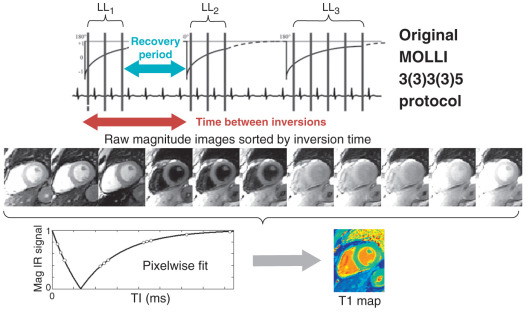
The MOLLI method uses an SSFP readout. The readout drives the IR to recover more quickly and reaches a steady state that is less than the equilibrium magnetization (M 0 ). The effect of the readout ( Fig. 2.2 ) is an apparent recovery time referred to as T1*, which is less than the actual longitudinal recovery time, T1, which is the desired tissue parameter. As a result of the influence of the readout, the IR curve follows a three-parameter exponential signal model, S (t) = A − B exp(− t /T1*), where t represents the inversion time and T1* is the apparent T1. The measured values may be fit to the three-parameter model to estimate A, B, and T1*, which may be used to approximate T1 ≈ T1* (B/A − 1). The derivation for the so-called Look-Locker correction factor (B/A − 1) is based on a continuous readout using fast low angle shot (FLASH). Despite the fact that the MOLLI uses a gated SSFP readout, the signal model behaves as a three-parameter model where the Look-Locker correction is reasonably effective at low readout excitation flip angles (FAs).
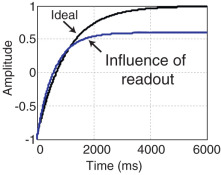
The analytic relationship between T1* and T1 for SSFP has been derived for continuous SSFP under somewhat idealized conditions such as ideal slice profile. Useful analytic derivations for gated SSFP with realistic slice profiles have not been developed due to complexity. Bloch simulations may be used to calculate the error inherent in this approximation and to gain insight into the sensitivity of various protocol parameters and design variables. The influence of various parameters such as T2, heart rate, off-resonance, and actual FA are referred to as confounders and are discussed later.
A number of modifications ( Table 2.2 ) to the original proposed MOLLI protocol have been proposed to shorten the acquisition duration or to improve the accuracy or precision. A shorthand nomenclature is used to label these protocols. The notation captures how many inversions (or saturations) are included in the experiment, how many images are acquired after each inversion, and how long the waiting period is between inversions. For example, a 3(3)3(3)5 protocol would indicate that there are a total of three inversions; three images are acquired (over three RR intervals) after the first inversion; this is followed by a waiting period of three RR intervals, and then three images are acquired, followed by another three RR waiting period; finally, there is a third inversion, after which five images are acquired. In an extension of this nomenclature, an “s” can be added to the intervals to indicate that images are acquired for a certain number of seconds and the waiting period is in seconds: that is, 5s(3s)3s would indicate two inversions with acquisition of images for at least 5 s, followed by a recovery of at least 3 s, and a second inversion with images acquired for at least 3 s. Because the number of electrocardiogram (ECG)-triggered images must be a whole number, the acquisition and recovery periods are rounded to the nearest multiple of the RR period to ensure an adequate duration. To avoid acquiring too few images for low heart rates (<60 beats per minute), the sequence never acquires fewer than the specified number of images: that is, 5 + 3 = 8 in this example. The recovery period is never less than the specified number of seconds. Acquiring and recovering with fixed minimum time periods helps gain independence of heart rate.
MOLLI | 3(3)3(3)5 | Messroghli et al. (original publication) |
3(3)5 | Ugander et al. and Salerno et al. | |
5(3)3 | Kellman et al. | |
4(1)3(1)2 | Schelbert et al. | |
2(2)2(2)4 | Salerno et al. | |
5(3s)3 | Kellman et al. | |
4(1s)3(1s)2 | Kellman et al. | |
5s(3s)3s | Kellman and Hansen | |
4s(1s)3s(1s)2s | Kellman and Hansen | |
ShMOLLI | 5(1)1(1)1(with conditional fitting) | Piechnik et al. |
SR is an alternative to IR that has gained renewed attention. Despite having a reduced dynamic range, SR has potential for improved accuracy. SR methods that use a saturation preparation for each measurement have the benefit that each measurement becomes independent of the others. By starting the recovery from a saturated state, the prior history is erased. Recovery periods between successive measurements are not required unless longer SR times are needed for fitting. Shown in Fig. 2.3 , the SASHA method uses SSFP readout very similar to the earlier SAP-T1 method, which used a spoiled gradient recalled echo (GRE) readout.
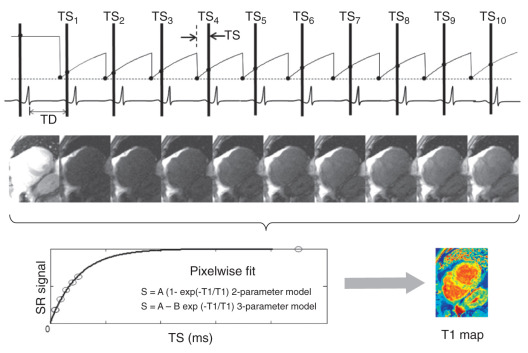
The SASHA method acquires multiple time points on the SR curve and does a pixelwise curve fit. To acquire a fully recovered image, an image is initially acquired before any saturation preparation: that is, starting from the equilibrium magnetization. Images are acquired on successive heartbeats using SR preparations with varying trigger delays. In the original proposed SASHA protocol, there are 10 images acquired at saturation delays uniformly spaced over the RR interval plus the initial fully recovered image, which serves as an important anchor point for the curve fit. The order in which the various delays are acquired is not significant for fitting, assuming ideal saturation. Importantly, the SR curve recovers as T1 and is not influenced by the readout so that it is not shortened to an apparent T1* < T1 as in the case of MOLLI. Therefore no correction is necessary, which eliminates the source of many inaccuracies of the IR-based MOLLI scheme. Because the readout does not lead to an apparent T1*, a higher FA readout is possible, which makes up for some of the lost dynamic range in using SR. The higher FA readout using SSFP with linear phase-encode ordering does slightly alter the shape of the recovery curve, causing an apparent bias: that is, the curve does not start at 0 for 0 delay. Thus the otherwise two-parameter signal model S (t) = A(1 − exp[− t /T1]) for SR assuming ideal saturation becomes a three-parameter model S (t) = A − B exp(− t /T1). The three-parameter model also absorbs any imperfection in the saturation efficiency attributed to the RF saturation pulse. However, the cost of estimating the additional parameter is loss of precision. Therefore there is a tradeoff between accuracy and precision in considering whether to use two- or three-parameter fitting. Although a center-out phase-encode order in which the center of k -space is acquired first has the potential to completely remove the influence of the readout, the use of center-out ordering with SSFP is problematic because of artifacts, and the use of center-out FLASH is associated with a significant loss of signal-to-noise ratio (SNR). An improved version of SASHA that uses variable flip angle (VFA) for SSFP readout minimizes the small bias attributed to a linear phase-encode order and therefore makes two-parameter fitting practical with negligible loss in accuracy. The SASHA VFA also has the benefit of improved transition to steady state, which greatly reduces ghost artifacts, particularly as a result of off-resonant fat, which was an issue with the original implementation.
The SASHA sampling scheme may be altered to acquire longer saturation delay measurements by allowing one or more heartbeat recovery periods between saturations. Measurements strategies that use recovery periods such as SMART1 may improve precision for specific ranges of T1 and heart rate but reduce the overall SNR efficiency somewhat. Optimized sampling strategies for SASHA are described for both two- and three-parameter fittings. Schemes that simply use a MOLLI strategy replaced with SR incur the problems of an apparent T1* without gaining the main benefits of SR. A combined IR/SR approach known as SAPPHIRE gains many of the benefits of IR and SR but still retains some of the problems associated with IR. Each method has its strengths and weaknesses in terms of accuracy, precision, and overall reproducibility.
ECV Mapping Methods
The ECV in the myocardium may be estimated from the concentration of extracellular contrast agent in the myocardium relative to the blood in a dynamic steady state. The ECV may be calculated as:
ECV=(1−hct)ΔR1myoΔR1blood=(1−hct)(1T1myo post−1T1myo pre)(1T1blood post−1T1blood pre)
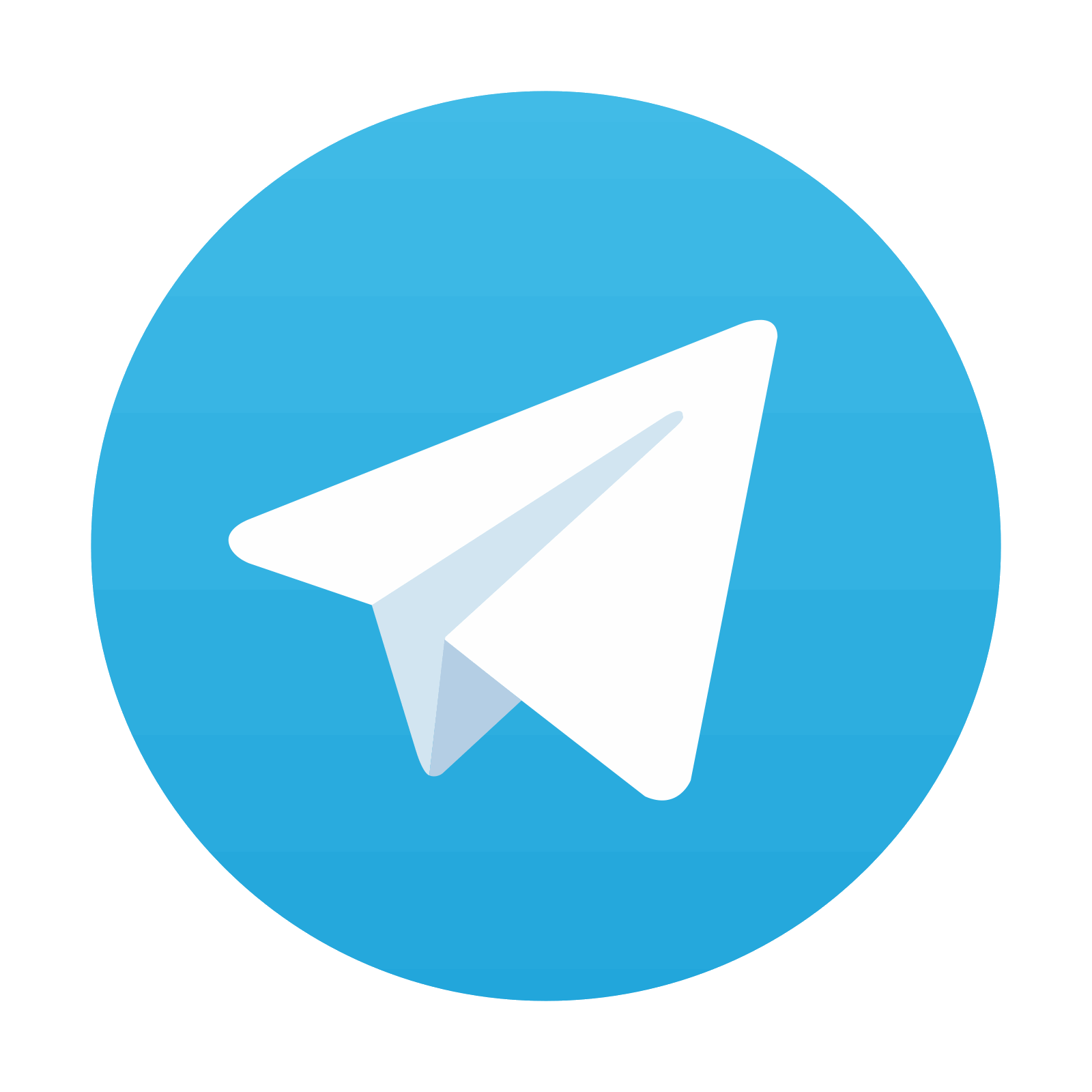
Stay updated, free articles. Join our Telegram channel
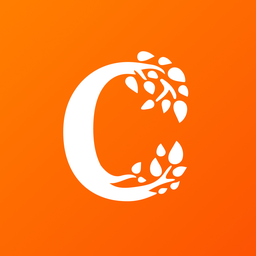
Full access? Get Clinical Tree
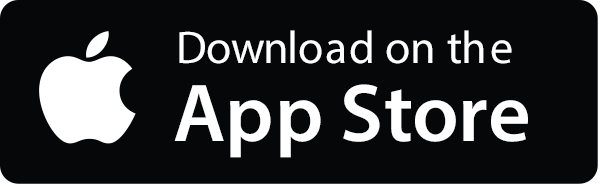
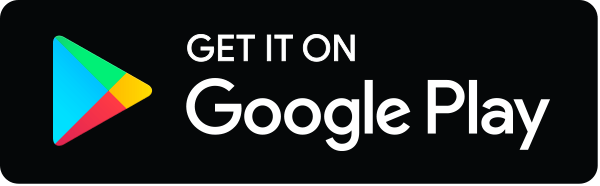
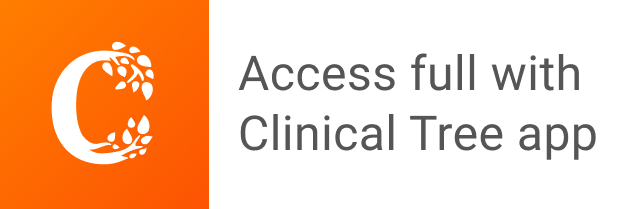