The Basic Principles of Dynamic Contrast–Enhanced Magnetic Resonance Imaging
Julio Cárdenas-Rodríguez
Xia Li
Jennifer G. Whisenant
Stephanie Barnes
Rudolf Stollberger
John C. Gore
Thomas E. Yankeelov
Introduction
Any tissue of the body that contains cells capable of division can develop cancer.1 The earliest detectable malignant lesions (cancer in situ) are often only a few millimeters in diameter and are commonly avascular during early stages. Cellular nutrition in these tumors depends on diffusion of nutrients, placing severe limitations on the size that such tumors can achieve.2 Avascular tumors are not detectable by magnetic resonance imaging (MRI) because their maximum diameter is only 150 to 200 μm.3 The transformation of a dormant tumor in situ into an invasive neoplasm is partially controlled by vascularization, and the rate of such transformation increases when the tumor itself promotes the formation of new blood vessels, a process known as angiogenesis.4,5 Because of multiple defects in the tumor-associated neovasculature,4 the vessels are characteristically leaky, fragile, and incompletely formed, leading to increased permeability relative to mature blood vessels in normal tissues that are the result of physiologic processes.6 Because angiogenesis is believed to be central to the development of nearly all solid tumors, the development of quantitative, noninvasive methods to characterize tumor-associated vessels is of great interest.
Dynamic contrast–enhanced magnetic resonance imaging (DCE-MRI) can quantitatively assess tumor-associated neovasculature and potentially determine how it responds to treatment. DCE-MRI requires the serial acquisition of images before and after the injection of a paramagnetic contrast agent (CA) so that the variation of MR signal intensity with time can be recorded for each image voxel or region of interest (ROI). As the agent enters into a tissue, it changes the native relaxation properties (T1, T2, and T2*) and, therefore, the measured MR signal intensity of the tissue to a degree that depends on the local concentration. After the agent is transported out of the tissue, the MR signal intensity returns to its baseline value. By analyzing the associated signal intensity time course using an appropriate mathematical model, physiologic parameters related to, for example, blood flow, vessel permeability, and tissue volume fractions can be extracted for each voxel or ROI.
DCE-MRI is actually an entire class of techniques, which can be separated into two basic subclasses: semiquantitative and quantitative methods. In the semiquantitative approach, parameters that characterize the shape of the enhancement curves are obtained and are typically related to the early contrast uptake, the maximum enhancement, the washout ratio, or the area under curve at, say, 60 seconds. Although it is relatively straightforward to obtain these data, a fundamental limitation of the approach is that these parameters are not directly related to underlying physiology. Conversely, the quantitative approach is based on fitting the enhancement curves to pharmacokinetic models to estimate physiologic parameters such as perfusion, permeability, and tissue volume fractions. Of course, quantitative methods are not without their own limitations and assumptions including the practical difficulties associated with the technique. This chapter will discuss the acquisition, analysis, limitations, and advantages of both semiquantitative and quantitative DCE-MRI studies.
Key Measurements for a Semiquantitative Dynamic Contrast–Enhanced MRI Study
Designing a DCE-MRI acquisition protocol requires a balance between temporal and spatial resolutions while achieving an appropriate signal-to-noise ratio (SNR). This balance is frequently dictated by the goals of the study as well as (practical) clinical demands. In general, coarser temporal resolution allows for obtaining a larger acquisition matrix over a fixed field of view (FOV), thereby enabling the acquisition of higher spatial resolution data. High spatial resolution is typically selected if a “semiquantitative” analysis is planned and probing tumor structural morphology and heterogeneity are viewed as central to experimental goals, as is the case in most clinical DCE-MRI studies where anatomic characteristics are critically important to the radiologist’s assessment. Conversely, coarser spatial resolution allows for the acquisition of higher temporal resolution data. Some high-temporal resolution DCE-MRI
studies acquire images as fast as 1 second,7 although most studies are acquired in the 10- to 30-second range.8 High temporal resolution is emphasized when a quantitative analysis is planned and the CA kinetics needs to be adequately sampled to allow for accurate model fitting.
studies acquire images as fast as 1 second,7 although most studies are acquired in the 10- to 30-second range.8 High temporal resolution is emphasized when a quantitative analysis is planned and the CA kinetics needs to be adequately sampled to allow for accurate model fitting.
In practice, the temporal or spatial resolution of a DCE-MRI protocol is determined by setting the parameters of a pulse sequence to different values. For example, increasing the number of phase-encoding steps leads to higher spatial resolution, lower SNR, and lower temporal resolution, whereas increasing the repetition time (TR) increases the SNR, lowers temporal resolution, and does not (directly) affect spatial resolution. Most MRI pulse sequences are categorized according to the method used to generate the radiofrequency (RF) signal (the echo) that is detected during an MRI scan. The two most common approaches for DCE-MRI studies are discussed in the following section.
Data Acquisition
Gradient-Echo Sequence
A gradient-echo (GE) sequence begins with excitation of the transverse magnetization in a slice of material. After the excitation, the polarity of the slice-selective gradient is reversed to refocus the spins in the slice. The excited slice is subsequently imaged using frequency and phase encodings. The pulse sequence, including excitation, phase encoding, and readout, is repeated M times with different values of the phase-encoding gradient. The repetition time (TR) is the time interval between consecutive excitations, so that the total scan time is given by M × TR. Thus, the spatial resolution (as determined by M) directly affects the temporal resolution as noted in the previous section. Please see Geek Box 1 for a mathematical description of the gradient-echo sequence.
Geek Box
The signal intensity equation for a gradient-echo image is given by:

where ρ is a constant describing proton density and scanner gain, α is the flip angle, T1 is the longitudinal relaxation time, T2* is the apparent transverse relaxation time, and TE is the echo time, which is the time interval between the center of the excitation pulse and the gradient echo. Because gradient-echo sequences do not have the refocusing pulse used in spin echo (see section about Rapid Gradient Echo Imaging), they are susceptible to additional losses above the normal T2 decay, which are manifested in the T2* term in Eq. [1].
Spin-Echo Sequence
The fundamental difference between gradient-echo and spin-echo pulse sequences is that the latter includes a 180-degree pulse that causes formation of a spin echo during signal acquisition. Consequently, spin-echo sequences are less sensitive to magnetic inhomogeneities than gradient-echo sequences as all the spins are refocused after the 180-degree pulse. Geek Box 2 provides a mathematical description of the spin-echo sequence.
As mentioned before, the analysis of DCE-MRI requires the acquisition of MR images (approximately) every 5 to 30 seconds after the administration of a contrast agent. The basic gradient-echo and spin-echo sequences described earlier can be optimized to achieve such temporal resolution, though only through two main ways (assuming the number of excitations is one): (a) decreasing the number of phase-encoding steps and (b) shortening the TR. In practice, the number of phase-encoding steps is controlled by the desired spatial resolution; therefore, rapid DCE-MRI is typically achieved by using very short TRs (usually on the order of 10 to 50 msec) or acquiring more than one echo on each phase-encoding step when a longer TR is used (100 to 250 msec). The strategies to perform rapid gradient-echo and spin-echo acquisitions are discussed next.
Rapid Gradient-Echo Imaging
Rapid gradient-echo imaging sequences can be divided into different two groups. The first group is composed of pulse sequences that use radiofrequency and gradient spoiling to destroy the transverse magnetization after each signal acquisition while also maintaining a steady-state longitudinal magnetization (so-called spoiled gradient-echo methods). The second group is composed of pulse sequences that maintain transverse and longitudinal magnetization in equilibrium (refocused gradient echo).
Spoiled Gradient-Echo Imaging
As mentioned previously, a very short TR (say, <50 msec) can be used to acquire MR images at very high temporal
resolution; however, when the TR is short and on the scale of T2, variations in the phase of magnetization from the production of stimulated echoes produce image artifacts. A way to reduce this effect is to destroy (i.e., spoil) the remaining transverse magnetization after each acquisition. (It is important to note, however, that gradient spoiling alone does not destroy the transverse magnetization as RF spoiling does; it only dephases the transverse magnetization.)
resolution; however, when the TR is short and on the scale of T2, variations in the phase of magnetization from the production of stimulated echoes produce image artifacts. A way to reduce this effect is to destroy (i.e., spoil) the remaining transverse magnetization after each acquisition. (It is important to note, however, that gradient spoiling alone does not destroy the transverse magnetization as RF spoiling does; it only dephases the transverse magnetization.)
TABLE 23.1 COMMON PULSE SEQUENCES USED FOR DCE-MRI AND THEIR NOMENCLATURE AS A FUNCTION of VENDOR | |||||||||||||||||||||||||||||||||||
---|---|---|---|---|---|---|---|---|---|---|---|---|---|---|---|---|---|---|---|---|---|---|---|---|---|---|---|---|---|---|---|---|---|---|---|
|
The basic two-dimensional (2D) spoiled GE (SPGE) sequence is usually known as FLASH (fast low-angle shot) or spoiled GRASS (gradient-recalled acquisition in the steady state), and its signal intensity is described by Eq. [1]. The name used by different vendors for SPGE sequences is shown in Table 23.1. An important feature of SPGE sequences is that because the excitation pulse is usually small (5 to 20 degrees) and the TR ≈ T2*, the contrast is mainly a function of T1 and spin density.
Refocused Gradient Echo
The goal of the refocused gradient-echo approach is to maintain the phases of all transverse components including stimulated echoes. Other names for this sequence are shown in Table 23.1. The main advantage of this pulse sequence is that the steady-state magnetization is relatively large even when the TR is extremely short (<20 msec). The signal intensity, however, is determined mainly by the ratio T2/T1, which makes it difficult to quantify the effect of a CA in a tissue of interest because T1 and T2 are simultaneously affected by the CA.
Fast Spin-Echo Imaging
The main strategy used for fast spin-echo (FSE) imaging is to use a series of 180-degree pulses to produce a train of spin echoes after each excitation. After each echo, the phase encoding is cancelled and a different phase encoding is applied to the following echo. Thus, more than one echo can be acquired in the same repetition (TR), which leads to a decrease in acquisition time equal to the number of echoes acquired after each excitation (echo train). This effectively reduces the number of excitations needed to acquire an image. For example, if the echo train is equal to four, the number of separate excitations is now reduced by a factor of four compared to standard SE sequence. A basic FSE sequence is usually referred to as a RARE (rapid acquisition with relaxation enhancement) or turbo spin echo (see Table 23.1).
Multiple Gradient-Echo Imaging
The strategy behind multiple gradient-echo (MGE) sequences is to acquire more than one echo during each TR. As in the standard GE, the MGE starts with excitation of the transverse magnetization, which is then frequency and phase encoded; these steps are repeated n times to create an echo train (like in FSE). The number of repetitions needed to acquire an image will depend on the number of phase-encoding steps and the echo train. The main difference between FSE and MGE is the exponential rate at which the signal decays during the echo trains; MGEs decay with T2* at a rate usually on the order of 5 to 60 msec, whereas the FSEs decay at rate of about 50 to 500 msec owing to a complex interplay of spin echoes, stimulated echoes, and higher-order echoes.
Echo Planar Imaging
The time needed to acquire an image depends on the repetition time (TR) and the number of phase-encoding steps (M). This time is M · TR for SE and GE sequences, whereas for FSE and MGE sequences the time is reduced by a factor equal to the echo train. The goal of the echo planar imaging sequence (EPI) is to collect the phase-encoding information for the entire image in a single TR. Thus, the time needed to acquire an entire image is equal to TR. To accomplish such a task the frequency- and phase-encoding gradients are varied in a way that allows sampling of all of k-space after a single excitation. The main limitations of this sequence are that its SNR is much lower than standard FSE and GE sequences because only one excitation pulse is used, and that the signal generated after the single excitation is very sensitive to imperfections in the magnetic field and decays exponentially in (typically) less than 50 msec.
Semiquantitative Analysis of Dynamic Contrast–Enhanced MRI Data
Semiquantitative analyses of DCE-MRI data are performed on the measured signal intensity or the calculated concentration of contrast agent time courses and aim to obtain descriptors of the tissue of interest based on visual inspection or enhancement curve analysis. The visual inspection method is based on a subjective evaluation of the signal intensity time courses, in which each curve is classified in accordance with a predefined evaluation system such as the one shown in Figure 23.1. The high-numbered curves are interpreted as representing more aggressive tumor enhancement kinetics. This method has shown good performance in differentiating malignant from benign tumors.9,10 Common parameters derived from the enhancement curves include the maximum enhancement ratio (MER), the time-to-peak (enhancement) (TTP), and area under the curve (AUC), which are illustrated in Figure 23.2. MER is the percentage increase of the signal between the baseline signal intensity (Spre) and the maximum signal intensity in the curve (Smax) and is defined in Geek Box 3. TTP is defined as the time lapse between the initial signal enhancement after injection and the point of maximum signal (Fig. 23.2). The slope is calculated by dividing MER by TTP. The initial area under the enhancement curve (iAUC) is calculated as the definite integral of signal intensity (or CA concentration in some studies) from the last precontrast time point to a fixed postcontrast time. The interval for which the area under the curve is calculated is described as iAUCtend, where tend denotes the last time point used in the integration (over signal or concentration; see Geek Box 4). The iAUC is usually reported from 0 to 60 or 80 seconds, that is, iAUCt60 and iAUCt80, respectively.11,12 The following sections will refer to iAUC without specifying the final point because different applications use different time intervals.13
Geek Box
The maximum enhancement ratio (MER) is defined as the percentage increase of the signal between the baseline signal intensity (Spre) and the maximum signal intensity in the curve (Smax):

These semiquantitative parameters are easily accessible with simple computer routines and have been successfully used to monitor cancer growth and treatment response (see, e.g., Fujimoto et al.11 and Jia et al.12). But they do not necessarily have a clear physiologic interpretation, as they are mixed measures of the tissue’s blood flow, vascular permeability, and fractional volumes. For example, the iAUC reflects tissue blood flow and vascular permeability, but it is also an indirect measure of the fractional interstitial space. Additionally, the degree to which each of these physiologic parameters contributes to the iAUC is at least partially determined by what time point is taken as the end (tend) of the initial portion of the enhancement curve.13 When signal intensity
measurements are used to compute these parameters, it can be quite difficult to compare scans performed at separate times and/or different institutions. A way to overcome this limitation is to quantify the absolute concentration of the CA and fit the concentration-activity curves to a pharmacokinetic model; such is the approach used in the quantitative analysis of DCE-MRI data.
measurements are used to compute these parameters, it can be quite difficult to compare scans performed at separate times and/or different institutions. A way to overcome this limitation is to quantify the absolute concentration of the CA and fit the concentration-activity curves to a pharmacokinetic model; such is the approach used in the quantitative analysis of DCE-MRI data.
Geek Box
The initial area under the curve (iAUC) is calculated as the definite integral of signal intensity or CA concentration time course from the last precontrast (baseline) time point to a fixed postcontrast time point:

where tinitial and tend are the beginning and ending times of the period for which contrast accumulation is to be estimated. (Please note that, in practice, Eq. [4] can be implemented as a summation rather than a definite integral.) The interval for which the area under the curve is calculated is described as iAUCtend, where tend denotes the last time point used in the integration (over signal or concentration).

DCE-MRI requires the acquisition of heavily T1-weighted images before, during, and after the injection of a paramagnetic contrast agent.
Although there are several commonly available pulse sequences that can achieve this end, spoiled gradient-echo approaches are typically selected.
Once the data are acquired there are several, easily performed semiquantitative analyses that have shown clinical value by characterizing enhancement curve shapes, which provide indirect estimates of various hemodynamic parameters.
Key Measurements for a Quantitative Dynamic Contrast–Enhanced MRI Study
The inputs required for the quantitative analysis of DCE-MRI data are the time variations of the absolute concentration of a CA within a tissue of interest (TOI) and in the blood plasma (the so-called arterial input function, or AIF). These two concentration versus time curves are then fit to a pharmacokinetic model to estimate physiologic parameters that describe the CA movement across the vascular endothelium and accumulation in the extravascular extracellular space (EES). The measured MRI signal intensity, however, is not typically linearly related to concentration, so the signal intensity cannot be directly converted to concentration of contrast agent. Thus, although similar data acquisition methods described earlier in the previous section are also employed in quantitative DCE-MRI studies, there are several subtle but critically important differences that allow for conversion from the MR signal intensity to CA concentration. The native T1 of the tissue(s) under investigation must be measured before injection (termed T10). Then a series of heavily T1-weighted images are acquired as described in the Data Acquisition section. The AIF and the CA concentration in the tissue ROI are then calculated using the observed changes in MRI signal and knowledge of T10. Finally, these two CA concentration curves are fit to a pharmacokinetic model.
Geek Box
In using a multiple-TR approach to measure T1 via a spin-echo sequence, a minimum TE is chosen so that TE ≪ T2 and the exp(−TE/T2) term in Eq. [2] goes to 1, leaving the signal intensity for this pulse sequence as:

where all parameters are as previously defined.
Measuring T1 in Tissue before Injection of a Contrast Agent
There are several methods for estimating precontrast T1 values at the voxel level using either spin-echo or gradient-echo pulse sequences.14 A spin-echo sequence can be repeated with a (minimum) constant echo time (TE) and variable TR in a method known as saturation recovery. (see Geek Box 5). The principle of this method is to collect a series of images with different TR values and fit the signal voxel by voxel to Eq. [5] to construct a T10 map (i.e., a map of T1 values before CA administration). Although this approach can yield accurate T1 values, it can lead to very long acquisition times and therefore is seldom used in clinical studies. An alternative, and frequently employed, method for measuring T1 is through the acquisition of multiple spoiled gradient-echo images at different flip angles.15 To calculate the T10 map, the signal intensities measured at each flip angle are fit to Eq. [6] to extract T1 at each voxel location (see Geek Box 6). This method drastically decreases the total measurement time (for large volumes of interest) compared to many other methods while still maintaining
the high SNR of those methods and has been used by many investigators.16,17 The method, however, does suffer from errors resulting from B1 inhomogeneity, a problem that is especially pronounced at field strengths greater than or equal to 3T.
the high SNR of those methods and has been used by many investigators.16,17 The method, however, does suffer from errors resulting from B1 inhomogeneity, a problem that is especially pronounced at field strengths greater than or equal to 3T.
Geek Box

where all parameters are as previously defined. (This is analogous to the simplification that happens from Eq. [2] to Eq. [5].) The signal intensity is measured at an array of flip angles and the resulting data are then fit to Eq. [6] to extract T1 at each voxel location. This, however, requires the flip angles played out to be known exactly. Errors in the transmit B1 field can lead the flip angles to be on error. Mapping B1 at higher field strength is highly recommended.
In practice, the method of obtaining the precontrast T1 map is frequently dictated by the demands of the study. In preclinical studies, where there is typically not the same time limitations found in the clinic, it is worthwhile to consider employing the longer—but more accurate—T1 mapping methods. Conversely, in the clinical setting, where time is critical, the multi–flip angle approach is commonly used.
Calculating Contrast Agent Concentration in a Dynamic Contrast–Enhanced MRI Experiment
A major difference between DCE-MRI data and other (non-MRI-based) DCE imaging methods is that DCE-MRI measures the effect of the CA on water relaxation and not the concentration of the contrast agent itself. Clinical DCE-MRI studies employ CAs based on gadolinium chelates, which shorten the tissue’s native T1 and leads to an increase in signal intensity (over a limited range of CA concentration) in T1-weighted images as described in Geek Box 7. Because images must be acquired very rapidly in a quantitative DCE-MRI study, there is not enough time to explicitly measure the R1(t) time course. Rather, R1(t) is estimated from either Eq. [1] or Eq. [2] (depending on whether the data are acquired with a GE or SE sequence, respectively) in conjunction with the T10 data. Eq. [7b] is then used to estimate CA(t). An example of how to calculate CA(t) for a spin-echo sequence is shown in Figure 23.3. The procedure for a gradient-echo sequence is analogous. An important assumption of this method is that the CA relaxivity (r1, in units of mM−1s−1) is the same for the ROI and AIF, an assumption that can be invalid in certain circumstances.18 The relationship used in Figure 23.3E., R1(t) = R10 + r1· [Gd(t)], assumes linearity, which may not always be true. The method
typically used to measure r1 for a given CA is to generate samples of known concentrations of the CA and acquire accurate T1 measurements (see, e.g., the previous section). Given the distribution of concentration of CA in the samples and their associated T1s, these data can then be first to the equation for R1(t) mentioned earlier to extract r1. More details on this process can be found in Chapter 6. Moreover, with higher gadolinium concentrations, T2 and T2* effects cannot be neglected.
typically used to measure r1 for a given CA is to generate samples of known concentrations of the CA and acquire accurate T1 measurements (see, e.g., the previous section). Given the distribution of concentration of CA in the samples and their associated T1s, these data can then be first to the equation for R1(t) mentioned earlier to extract r1. More details on this process can be found in Chapter 6. Moreover, with higher gadolinium concentrations, T2 and T2* effects cannot be neglected.
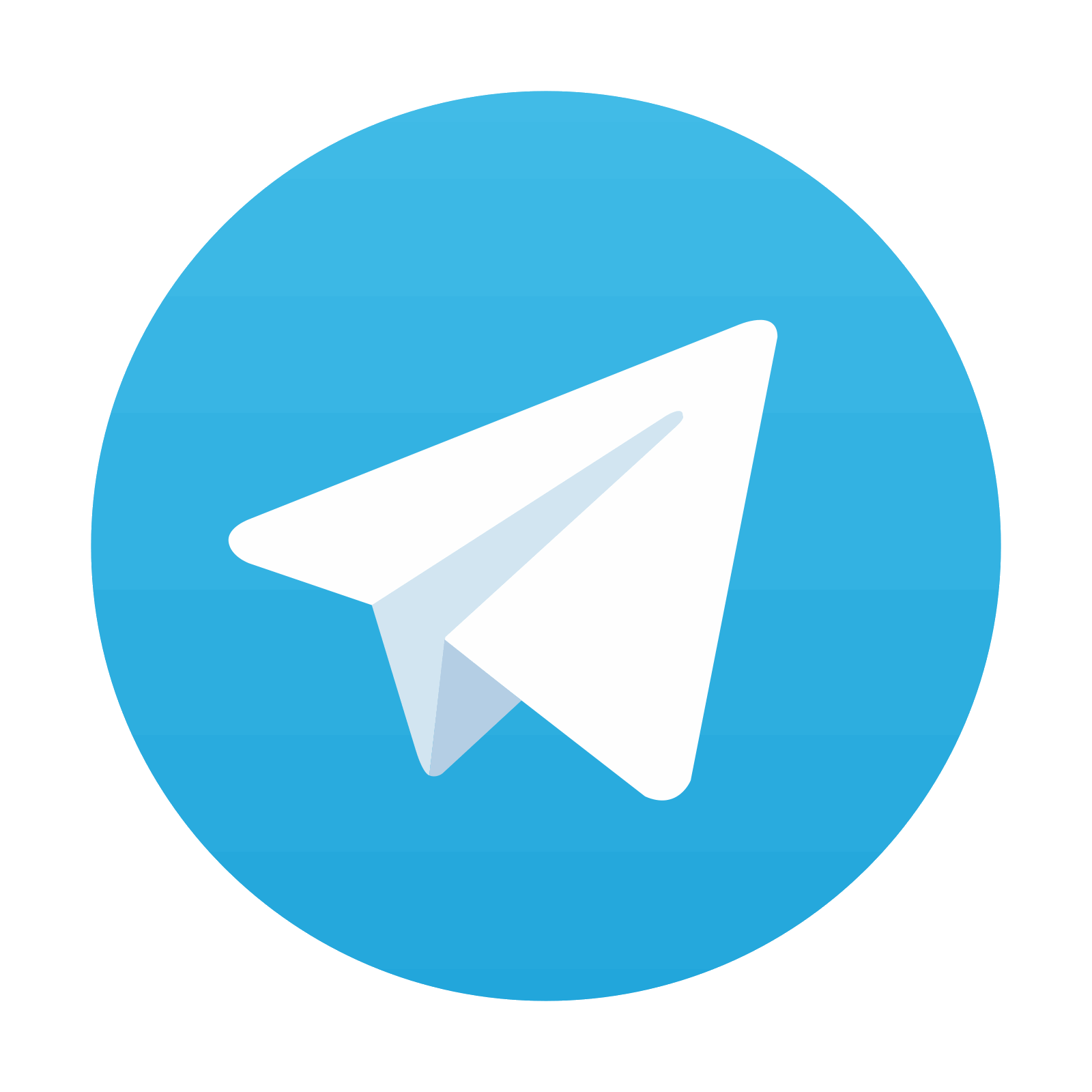
Stay updated, free articles. Join our Telegram channel
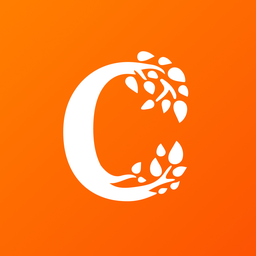
Full access? Get Clinical Tree
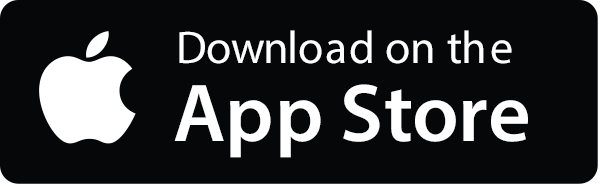
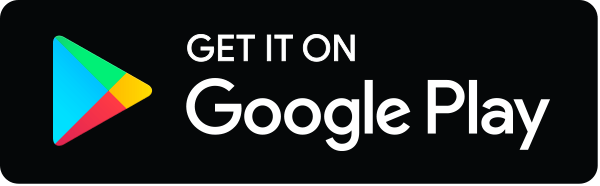