Fig. 9.1
Normal MDCT vascular mapping of the peripheral arteries. Coronal MIP images were obtained in a 39 year old male with a thigh mass which demonstrate patent and normal caliber peripheral arterial tree, well visualized in the thigh and calf regions. MDCT provides excellent spatial resolution with clear depiction of small branch vessels of the profunda femoris and popliteal arteries
Applications of Dual Energy in Peripheral Arteriograms
Bone subtraction is important for optimal post-processing of MIP and VR images and has been the most extensively evaluated application of dual energy imaging in the peripheral arteries. Accurate bone subtraction is a prerequisite to high quality 3D visualization in the periphery. Given that both intravascular contrast and bone demonstrate high attenuation on CT images, in the absence of postprocessing, overlying bone obscures visualization of the complete vascular tree, which is particularly problematic in vessels below the knee (Fig. 9.2) and in the forearm. Bone subtraction techniques with single-energy imaging identify bone using threshold-based, region growing algorithms which are prone to inadvertent arterial subtraction, particularly in vessels closely apposed bone, due to overlap in CT attenuation values. This can result in post-processed MIP and VR images with artifactual vessel occlusions (Fig. 9.3). Dual energy, which allow separation of the iodine and bone pixels based on differences in x-ray absorption at two energy levels, provide improved and subtraction of bone compared single energy techniques [7, 8]. In addition to bone, calcified plaque can obscure the vessel lumen on 3D MDCT images, making comprehensive assessment of stenosis challenging [9, 10]. Dual energy techniques have the ability to perform automatic plaque calcified plaque subtraction which may improve lumen visualization the setting of large caliber, heavily calcified vessels (Fig. 9.4) [11, 12].
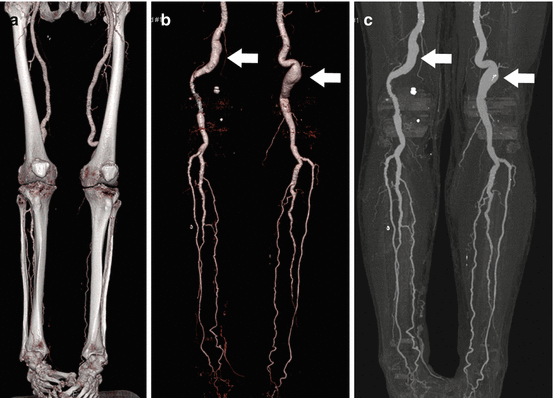
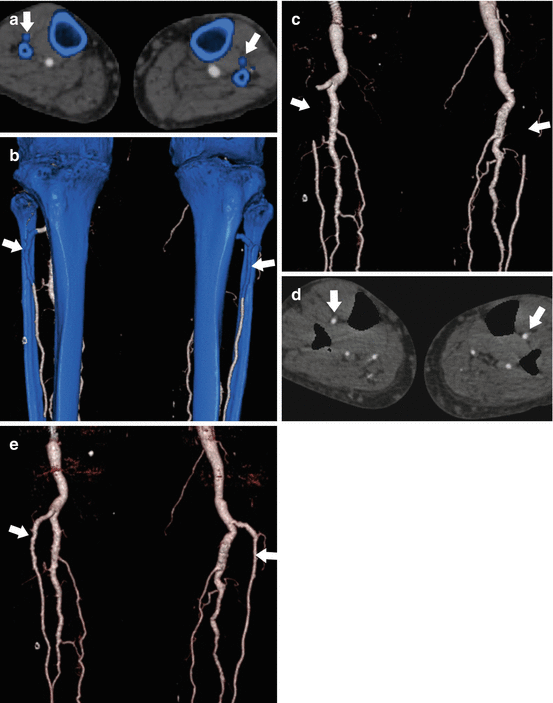
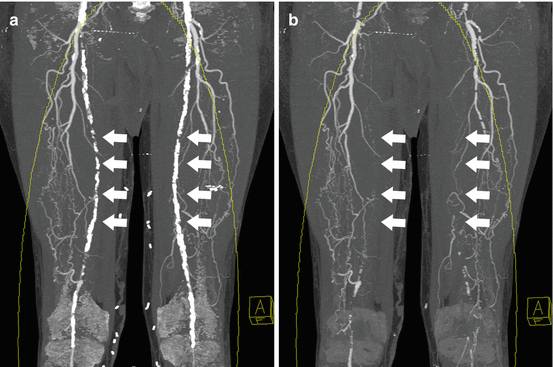
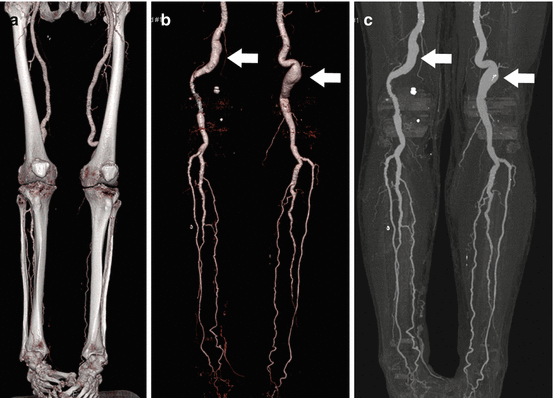
Fig. 9.2
Optimized visualization of peripheral CT angiography datasets using bone removal. (a) Coronal volume rendered images from a CT angiogram of the lower extremities obtained in a 63 year old male with Loeys-Dietz syndrome demonstrate ectatic and tortuous superficial femoral arteries, however, the popliteal arteries and calf vessels are not visualized due to overlying bone. Bone-subtracted coronal volume rendered (b) and MIP (c) images provide a complete assessment of the peripheral vascular tree on a single image, similar to that seen with digital subtraction angiography
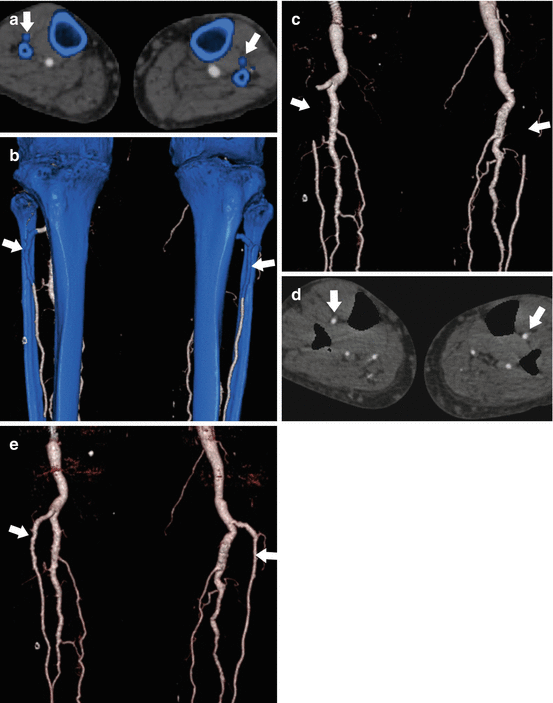
Fig. 9.3
Limitations of standard single energy bone subtraction techniques. Axial MPR (a) and coronal VR (b) images from the same 63 year old male with Loeys-Dietz syndrome. Using a standard single energy threshold-based automated bone subtraction technique, bone pixels are highlighted with blue. There is inadvertent labeling of the proximal anterior tibial arteries as bone bilateral (arrows). This results in segmental non-visualization on a coronal subtracted VR image (c) which mimics occlusion. Dual energy bone subtraction accurately identifies the anterior tibial artery as vessel and not bone (d), resulting in increased accuracy of the VR image (e) without the potential for misdiagnosis
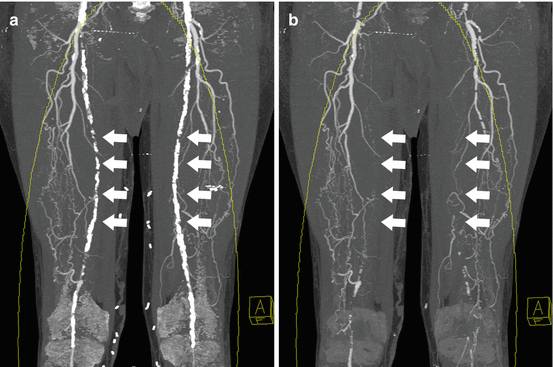
Fig. 9.4
Dual energy plaque subtraction. (a) Coronal MIP images from a CT angiogram obtained in a 69 year old male with extensive peripheral arterial disease demonstrate extensive severe calcification of the bilateral superficial femoral arteries that obscures visualization of the lumen on MIP images (arrows). (b) With dual energy plaque removal, the calcified plaques are removed, providing a more clear demonstration of bilateral SFA occlusions, which were confirmed on axial source images
Dual energy imaging offers additional potential advantages beyond bone and iodine discrimination through the use of virtual monoenergetic reconstructions. Standard single energy CT examinations use a polychromatic x-ray spectrum that includes a mix of photons with various energy levels, ranging from approximately 30–120 keV [13]. Dual energy post-processing can be used to estimate the expected pixel-based attenuation levels for a given imaging volume for imaging with a virtual monoenergetic beam, which can be reconstructed over a wide range of keV values from 40 to 190 keV. Virtual monoenergetic reconstructions in the 60–70 keV range have the potential to improve image contrast and overall image quality with similar or reduced noise profile compared to standard polyenergetic techniques [14, 15]. Higher energy reconstructions around 120 keV or greater may limit streak artifacts from metal prostheses [15]. Some authors have suggested these properties could allow for more reliable imaging in challenging patients, such as those that require reduced contrast dose due to renal insufficiency, reduced injection rates because of limited intravenous access, or those with metal prostheses.
Physics of Dual-Energy CT Peripheral Arteries
Several methods have been used for creation of dual energy studies, which differ depending upon the CT vendor. The most widely studied and first available method is dual source imaging (Siemens Healthcare) [16]. Both first generation (Siemens Definition) and second generation (Siemens Definition FLASH) scanners with dual energy capability have been widely available. In this system, dual energy information is provided through the means of two separate x-ray tubes and detectors that are angled at 90° from each other within the CT scanner. Image data is acquired simultaneously at two energy levels, either 80 and 140 kV (first generation) or 100 and 140 kV (second generation). Due to size limitations, the field-of-view for dual source dual energy imaging limited to the smaller of two detectors, which measures 26 cm in first generation scanners and 33 cm in second generation scanners. With the second generation scanner, a tin filter was introduced for the 140 kV x-ray source, which reduces the number of low energy photons and increases spectral separation between energy levels [13]. This also allows for acquisition of the lower energy data at 100 kV, rather than 80 kV, which improves noise profile, particularly in large patients [13]. Several types of single-source dual energy options have been developed or are under development from other CT vendors. Rapid-kV switching single source dual energy (CT750HD, GE Healthcare) uses an x-ray source that switches between two energy levels rapidly during the examination with both sets of imaging data acquired on the same detector, which has the advantage of no field of view restrictions [17]. A single source dual energy alternative using sequentially acquired low and high kV scans is also available (Aquilion One, Toshiba); although at the expense of increased radiation dose and risk of motion degradation due to duplicate scanning. Finally, sandwich-type single source dual energy detectors that utilize a superficial layer of low energy absorbing detectors and a deep layer of high energy detectors are under development and will likely become clinically available in the near future (IQon, Phillips Healthcare).
Once dual energy exams are acquired, whether with single or dual source scanners, postprocessing steps must be performed to extract tissue specific information from the imaging data. Using a process referred to as material decomposition, differences in x-ray absorption between the low and high tube voltage images are analyzed on a per voxel basis (Fig. 9.5). Through mathematic modeling, images can be separated into basis materials, due to differences in photon absorption at each spectra. In the case of automated dual energy bone subtraction, the most widely used application of dual energy imaging in the peripheral vasculature, voxels are evaluated for the presence of calcium and iodine [18]. Iodine has significantly greater x-ray absorption at low energy levels than calcium due to a strong photoelectric effect, resulting in higher attenuation on images from the low energy spectra. Calcium has a weaker photoelectric effect, resulting in relatively less change between high and low spectra. Post-processing software uses these differences to identify the relative amount of calcium and iodine contained in each voxel [8]. Voxels that contain calcium above a threshold level can be automatically identified and removed, creating a bone subtraction mask. The result is automated rapid bone removal with a single-click postprocessing step, which holds significant advantages over the standard threshold-based region growing algorithms used for single source imaging that require time intensive manual correction. The same material decomposition process can also be used to synthesize virtual monochromatic dual energy CT data sets [19]. Using mathematical models, a mass density map of basis materials are estimated for each voxel. Monochromatic images are synthesized after calculating the expected photon absorption for the pre-specified energy level. These images can be created over a wide range of energy levels which can allow selection of the optimum keV for the optimum balance of contrast and noise.
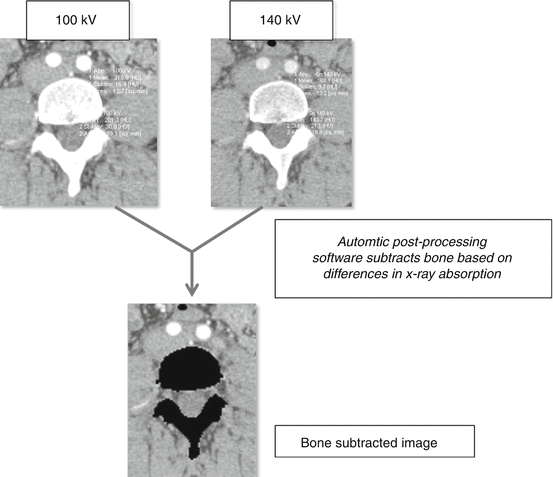
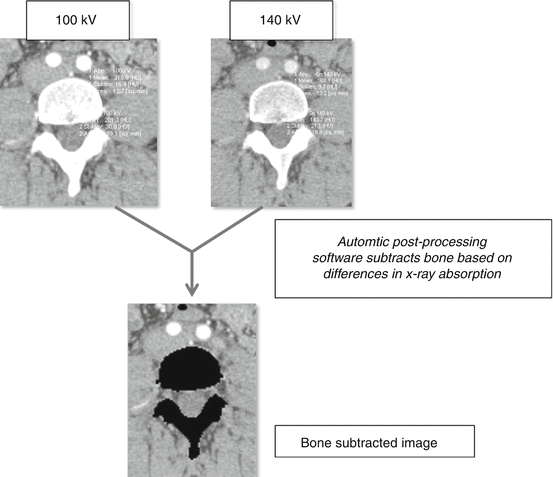
Fig. 9.5
Principles of dual energy bone subtraction. Dual energy CTA exams acquire images at two separate kVp (100 and 140 for this example). The relative differences in absorption between low and high kVp are different for calcium and iodine (calcium – 201/144 HU between low and high kVp; iodine 319/168), with calcium exhibiting a greater increase in HU at lower energy levels. Automatic post-processing algorithms use these differences to mathematically determine iodine and calcium content on a per voxel basis. This data is used to create bone subtracted datasets
Radiation dose for dual source, dual energy imaging is generally the same as single source examinations performed at 120 kV given that dose is divided between the two x-ray sources [20]. As a newer technology, the radiation dose implications for single source, rapid-kV switching dual energy imaging have been less rigorously evaluated. In an initial study by Pinho et al. examining the use of single-source dual-energy CT for evaluation of the abdominal aorta, radiation dose was significantly higher for dual-energy CTA acquisitions compared to single energy exams [17]. This is due at least in part to a limitation of current single source technology that prevents use of tube current modulation during dual-energy mode. However, total cumulative dose for dual energy exams is substantially lower single energy if unenhanced scans can be eliminated through creation of virtual non-contrast images.
Acquisition Protocols
Detailed first and second generation dual source and rapid-kV switching single source scan protocols for the upper and lower limbs are presented in Table 9.1. Injection protocols for dual energy runoff examinations are similar to those used for single energy examinations, with 100–140 mL of iodinated contrast administered through a large bore IV, ideally 18 gauge, at high injection rates, typically 4–5 mL/s, followed by a 40–50 mL saline flush. For abdominopelvic angiography and runoff through the lower limbs, a bolus tracker at the level of the mid abdominal aorta is used for triggering, set at 230 HU. If only the lower limbs are imaged, then bolus tracker can be placed in the popliteal artery. For upper limb CT angiography, the patient is positioned with both hands over the head, “superman” style (Fig. 9.6). The bolus tracker is placed at the level of the ascending aorta and triggered at 230 HU. For first generation dual source scanners using 140/80 kV, 1.2 mm collimation has been advocated by some authors due to improved noise profile for the 80 kVp acquisition [21]. However, with second generation dual source scanners utilizing the combination of Tin-filtered 140 and 100 kV imaging, thin, 0.6 mm collimation is available due to reduced noise at 100 kV. For first generation dual-source examinations, it may be necessary to bind and elevate the patient’s legs so that the lower extremities are included within the smaller 26 cm FOV.
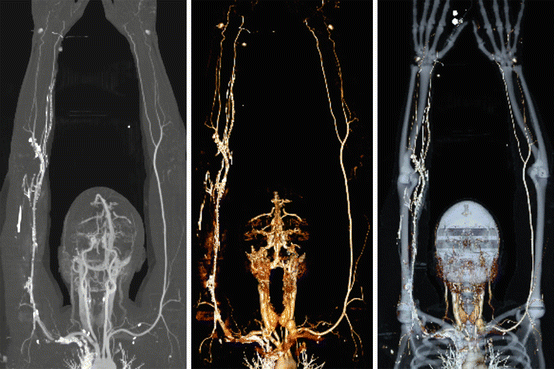
Table 9.1
Dual energy CT protocols
Technical parameters | Siemens definition flash dual source CT 128-MDCT scannera | Siemens definition dual source CT 64-MDCT [14] | GE discovery CT750HD CT 64-MDCT [17] |
---|---|---|---|
kVp | 100/140-Sn (Tin filtered) | 80/140 | 80/140 |
mAs | CareDose Dose 4D; reference mAs 250/193 | CareDose Dose 4D; reference mAs 80/440 | 600 |
Collimation | 32 × 0.6 mm | 14 × 1.2 mm | 64 × 0.6 mm |
Pitch value | 0.8 | 0.6 | 1.4 |
Scan direction | Craniocaudal (lower) | Craniocaudal (lower) | Craniocaudal (lower) |
Caudalcranial (upper) | Caudalcranial (upper) | Caudalcranial (upper) | |
Reconstructions | 1.5 × 1.0 mm | 1.5 × 1.0 mm | 1.5 × 1.0 mm |
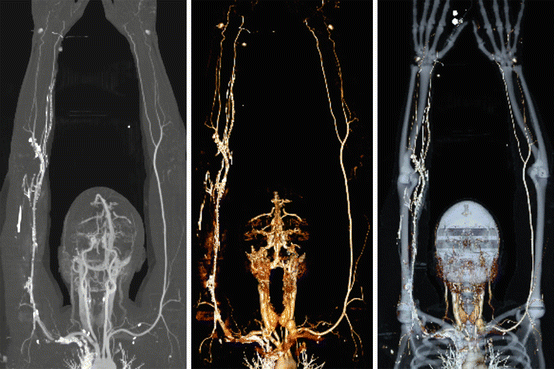
Fig. 9.6
Normal upper extremity CT angiogram with dual energy subtraction. Coronal MIP (left) and VR with (middle) and without (right) bone subtraction images from a patient with suspected upper extremity thrombus show a normal upper extremity runoff. Bilateral arms up positioning is preferred if patients can tolerate to improve image quality due to decreased noise from bones of the thorax. It is important to inject opposite the side of the extremity of concern to avoid streak artifact that might obscure the area of interest
Evidence for Dual Energy Bone Removal
Several studies have evaluated the use of automated dual energy bone removal for post-processing of peripheral CTA datasets in patients evaluated for PAD. To date, the majority of studies have been performed first generation, dual-source dual-energy CT scanners. To date, no published studies evaluating use of dual energy CT in the upper limbs are available.
Initial studies compared dual energy to traditional threshold based bone subtraction techniques, the first of which was published in 2008 by Meyer et al. [7]. Dual energy peripheral CTA was performed in 50 patients with automated post-processing performed using standard, threshold-based manual bone subtraction techniques and automatic, dual energy based bone subtraction. Readers compared the speed, effectiveness of bone subtraction and extent of erroneous vessel erosions for each technique. Plaque subtraction was also evaluated separately, and will be discussed in the section below. The authors found that dual energy bone subtraction was significantly faster than manual techniques (2 min vs. 7 min) and resulted in less compromising vessel erosions (11 % vs. 15 %), particularly for the arteries of the calf. However, compromising erosions remained common even with dual energy for both the anterior tibial (64 %) and dorsalis pedis (51 %) arteries. Dual energy studies had the disadvantage of greater amounts of residual non-subtracted bone, most often ribs and patella located outside of the smaller dual energy FOV. However, even with additional manual correction of residual bone, dual energy techniques remained substantially faster than traditional subtraction methods. A similar study from Sommer et al. in 2009 evaluated 51 patients with dual energy peripheral CTA and also compared speed and accuracy of manual and dual energy bone subtraction methods [8]. Their results closely mirrored those of Meyer et al., showing significant time savings with dual energy (3 min vs. 6 min) and significantly fewer vessel segmentation errors. The anterior tibial artery was the most commonly negatively affected vessel in both manual and dual energy methods.
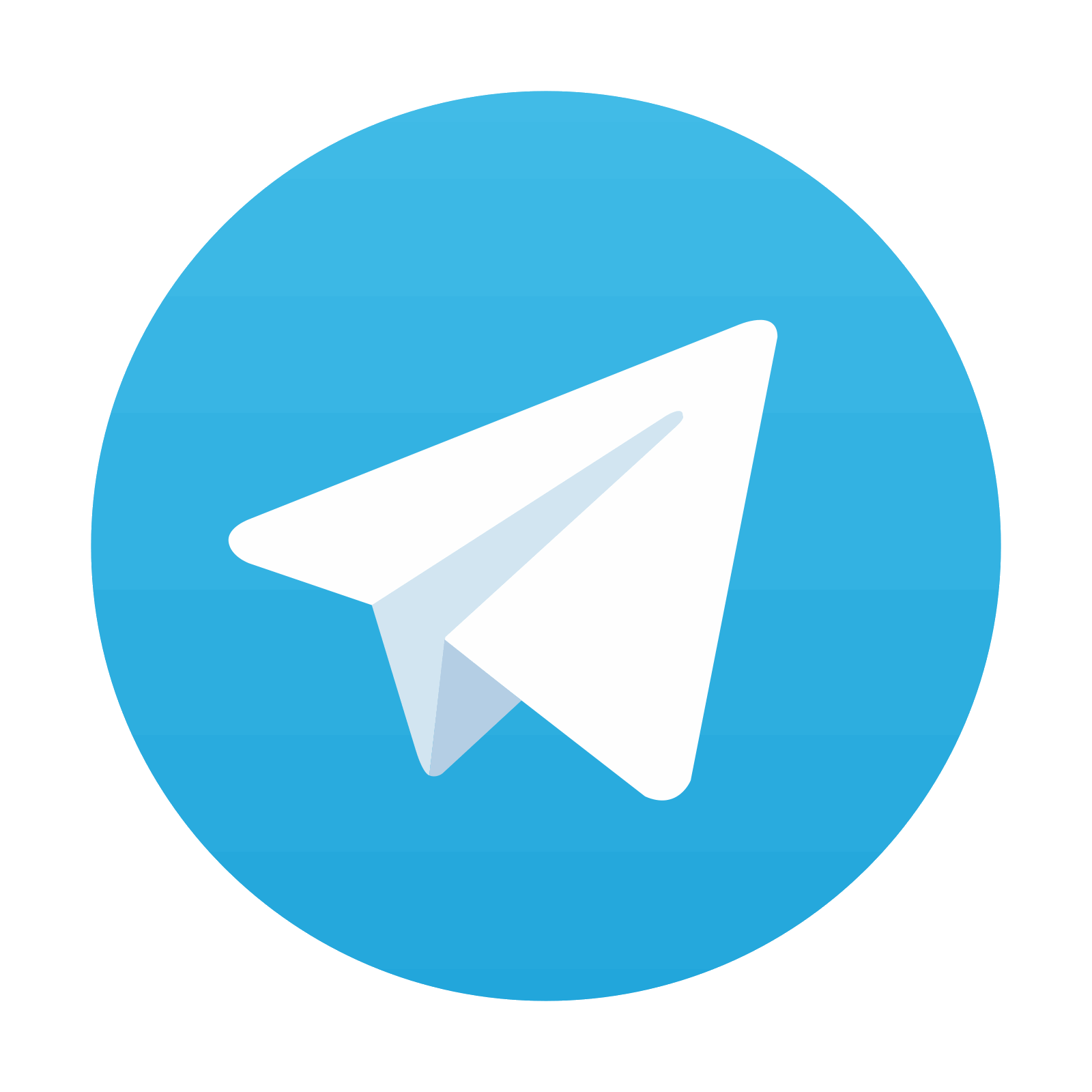
Stay updated, free articles. Join our Telegram channel
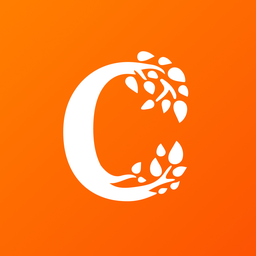
Full access? Get Clinical Tree
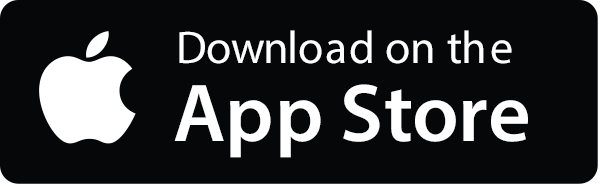
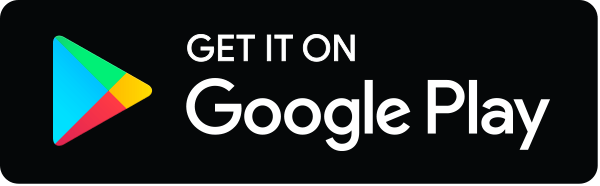