Fig. 15.1
Top, from left to right: T1-weighted, T2-weighted, proton density and FLAIR images, obtained in an MS patient. Bottom: same images overlaid with expert manual delineation of MS lesions (Data from the “MS longitudinal lesion segmentation challenge”, ISBI 2015; training subject 02, time point 01.)
Gadolinium–enhanced T1–weighted MR images: “active” lesions taking up contrast material and indicating inflammation and breakdown of the blood–brain barrier; the presence of enhancing lesions indicates ongoing disease activity, since only new lesions (under 6 weeks old) enhance (see Fig. 15.2).
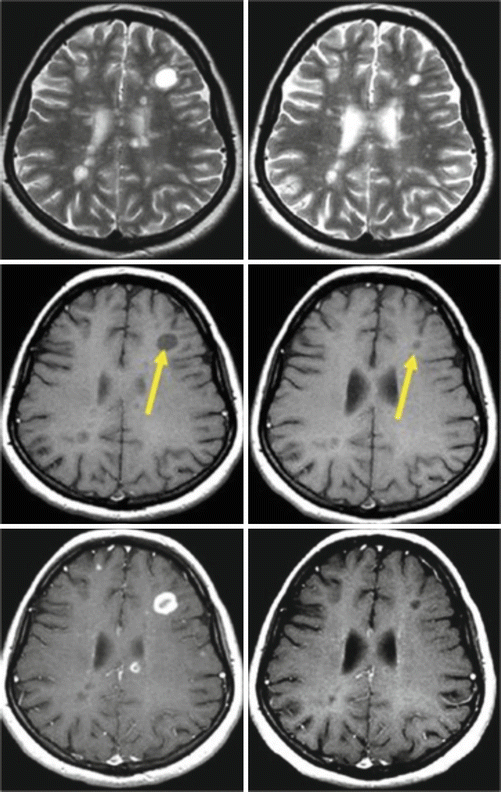
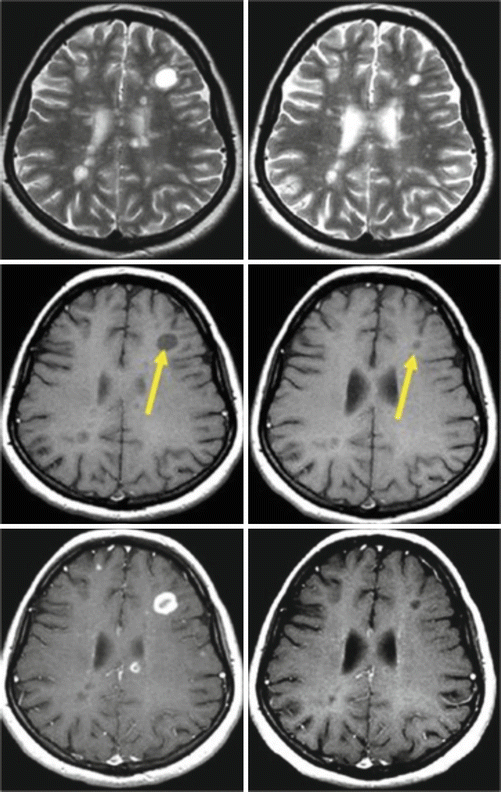
Fig. 15.2
Contrast enhancement in MS (Figure 5 from [45]; original caption: Serial magnetic resonance imaging (MRI) scans obtained in a patient with relapsing–remitting multiple sclerosis. T2–weighted (upper row), unenhanced T1–weighted (middle row) and contrast–enhanced T1–weighted (lower row) MRI scans obtained at baseline (left) and 1 year later (right). Observe the active ‘black hole’ in the subcortical white matter of the left frontal lobe (arrow), which shows a ring–enhancement pattern of contrast uptake. After 1 year, the lesion decreased in size (arrow), but remained hypointense on T1–weighted images, indicating an irreversible black hole). (Adapted from Reprinted by Permission from SAGE Publications, Ltd.: Ther Adv Neurol Disord. 6(5):298–310, copyright 2013
T2–weighted MR images, fluid attenuated inversion recovery (FLAIR) MR images and proton density (PD) MR images: on these imaging sequences with a long repetition time (TR), lesions appear as hyperintense spots compared to the surrounding brain parenchyma (see Fig. 15.1).
The “lesion load”, defined as the total volume of lesions in the brain, is one of the most important biomarkers in MS. Often, a distinction is made between T2 lesions (i.e. lesions that appear hyperintense on T2-weighted or FLAIR images), T1 lesions (i.e. lesions that appear hypointense on T1-weighted images, the so-called black holes), as well as contrast-enhancing lesions.
In addition to the lesion load, brain volumetry [25] and, more specifically, cerebral atrophy [5] and, in particular, grey matter (GM) atrophy [23] are currently considered to be essential biomarkers, since they are correlated with the speed of disease progression (see Fig. 15.3). Thus, apart from the detection of lesions, quantification of brain volumes and atrophy rates is increasingly important in the management of patients with MS.
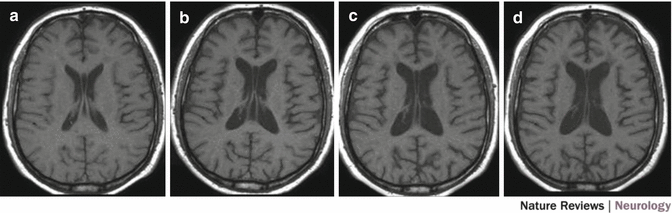
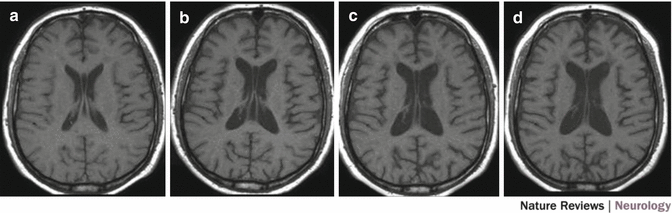
Fig. 15.3
Brain atrophy in an MS patient (Figure 2 from [60] with original caption: (a) Baseline scan. ( b–d) Regular scans over a 6–year follow–up period. Disease progression can be seen in the form of the increasing size of ventricular and subarachnoid spaces. These changes reflect brain volume loss over time, indicating progressive neurodegeneration). (Adapted from Reprinted by permission from Macmillan Publishers Ltd: Nature Reviews Neurology 11, 597–606, copyright 2015
15.2.2 Natural Course of the Disease
From a clinical point of view, MS starts with a first attack or a “clinically isolated syndrome” (CIS) suggestive of MS. CIS patients with a normal cerebral MRI at presentation have only a 5 % risk of subsequent clinical attack and thus of progression to clinically definite MS in the next 1–5 years [3]. Conversely, patients with cerebral lesions on MRI have a considerably higher risk, although the risk remains below 50 % when the total lesion volume does not exceed 1.2 ml [3].
When the disease evolves, it may take one of several forms: (1) relapsing-remitting MS (acute attacks are followed by remission periods), (2) primary progressive MS (steady worsening of neurologic functioning without any distinct relapses) and (3) secondary progressive MS (can follow relapsing-remitting MS and is characterised by a sustained build-up of disability, independent of any relapses). MR studies have confirmed the occurrence of lesions and the development of brain atrophy in all the MS types [14, 24, 29, 40].
Clinical evolution of MS is characterised by both motor and cognitive degradation [7, 41]. Pathological changes in the normal appearing white and grey matter are better correlated with progressive cognitive deficits than with visual, sensory and motor symptoms [4, 24, 51]. Brain atrophy, defined as the decrease in brain volume over time, is recognised as a typical consequence of MS [5]. The rate of brain volume loss in patients with MS exceeds the rate observed in healthy controls up to a factor of 2–8, that is, 0.5–1 % per year in MS patients versus 0.1–0.3 % per year in age-matched healthy controls [24, 48]. Formerly, it was believed that MS was defined pathologically as an inflammatory process confined to the white matter (WM). Nowadays, we know that in addition to white matter lesions, MS is also characterised by grey matter lesions and atrophy [15]. Moreover, MRI-based volumetric data have shown that grey matter atrophy better correlates with physical and cognitive disability than WM atrophy and T1 and T2 lesions [23, 24, 34].
Investigators have examined, and then confirmed, the unwritten rule that five new lesions (compared to the baseline MRI scan of the MS patient) are correlated with a higher risk of subsequent relapses [36]. A 10-year follow-up study on patients with relapsing-remitting MS confirmed the long-term clinical relevance of brain lesion evolution by showing that an accelerated clinical disability is particularly well correlated with the increase in T1 lesions [26]. Annualised lesion volume growth of 0.25 ± 0.5 mL (+6.7 ± 8.7 %) for T2-weighted lesions and +0.20 ± 0.31 mL (+11.5 ± 12.3 %) for T1-weighted lesions was established over a period of 10 years [26]. In a 20-year follow-up of 107 MS patients, lesion growth was 0.80 mL/year in those who were relapsing-remitting but was 2.89 mL/year in secondary progression [18]. In another follow-up study comparing progressive and nonprogressive MS patients over 10 years, it was found that GM atrophy is a good candidate as a disease progression biomarker [30]. In addition, brain atrophy and lesion load have been shown to be correlated with long-term disability in a multicentre 10-year follow-up study [39]. Table 15.1 provides a summary of these investigations.
Table 15.1
Evidence for the relevance of lesion and atrophy biomarkers in MS
Biomarker | Findings | Study population | Reference |
---|---|---|---|
T2 lesions | Annualised change: +0.25 ± 0.5 mL | 10-year follow-up RRMS | [26] |
T1 lesions | Annualised change: +0.20 ± 0.31 mL | Idem | Idem |
T2 lesions | Annualised change: +0.80 mL/year | 20-year follow-up RRMS | [18] |
T2 lesions | Correlated with disability progression | 10-year follow-up | [39] |
Total brain atrophy | Correlated with disability progression | Idem | Idem |
Total brain atrophy | CIS = −0.40 % ± 0.47 %, RR = −0.49 % ± 0.65 %, SP = −0.64 % ± 0.68 %, PP = −0.56 % ± 0.55 % | >1-year follow-up 963 subjects | [12] |
GM atrophy | Significant differences between progressive and nonprogressive | 10-year follow-up | [30] |
15.2.3 Treatment
MS researchers throughout the world acknowledge that, in addition to its well-established diagnostic value, MRI has an essential role in monitoring disease progression and therapeutic efficacy [2, 20]. Recent treatments, especially for the early stages of MS, focus on modifying the natural course of the disease and do not merely treat symptoms. Some of the more aggressive disease-modifying therapies can have serious side effects and are therefore not prescribed as first-line treatments. Criteria for switching from one treatment to another are still under active investigation. MRI-based monitoring of therapeutic effects becomes more and more essential in clinical trials and also in clinical practice. However, MRI-derived metrics are not yet standardised and still under development [2, 20, 27, 44].
In patients developing three or more active MRI lesions, in addition to a clinically active disease (relapses and disability progression), a change in treatment strategy is recommended [44]. During the course of disease-modifying therapy, new or enlarging lesions should be monitored on scans every 6 months to assess for change [43]. The presence of one or more Gd-enhancing lesions on a 6- or 12-month follow-up scan, or two or more new or enlarging lesions on a 12-month follow-up scan, should prompt consideration of therapy change [43].
Many lessons have been learned from clinical trials, for instance, that using lesion count and brain atrophy as endpoints might be more efficient than the Expanded Disability Status Scale (EDSS) [34]. Placebo-controlled trials in secondary progressive MS patients would require 32 subjects per arm to detect a 50 % treatment effect at 80 % power over 2 years, if MRI measures of brain atrophy (using the SIENA method [56]) were used as outcome measures [1]. Using EDSS as outcome measure, placebo-controlled trials would require about 150 patients per study arm to demonstrate statistically significant therapeutic effects for a study of 2–3 years [47].
The debate is ongoing whether whole-brain atrophy should be used as the gold standard for effective treatment of MS after the first year of treatment [48] or not [16]. A confounding factor is that whole-brain atrophy after 1 year of treatment might be an inaccurate parameter, due to the occurrence of pseudo-atrophy: the early reduction of brain volume as a result of a decreased inflammatory profile, rather than of the underlying disease [11]. Measuring GM atrophy, instead of whole-brain atrophy, is potentially more useful since pseudo-atrophy appears to affect white matter more than grey matter [48] and may persist for more than 2 years after treatment initiation.
Some clinical trials have shown that brain volume loss (or GM loss) is a good predictor for the natural course of the disease [11]. However, when disease-modifying treatments (DMTs) are used, conflicting results have been observed in various clinical trials. Differences in the mechanism of action of the drugs, the patient populations, the quality of the MRI scans and the software packages used for the analysis could explain the occasional discrepancy between these results.
15.3 Acquisition Requirements
Widespread application of MR imaging biomarkers is hampered by issues such as non-standardised imaging protocols, imaging artefacts, lack of normative data and manual segmentations to interpret values in clinical practice. In order to mitigate such issues and promote MR imaging in MS clinical practice, the MAGNIMS study group published guidelines for the use of MRI in MS diagnosis [46], as well as recommendations to improve imaging and analysis of brain lesion load and atrophy in longitudinal MS studies [59, 60]. The group recommends that “images should be acquired using 3D pulse sequences, with near-isotropic spatial resolution and multiple image contrasts to allow more comprehensive analyses of lesion load and atrophy, across time points. Image artifacts need special attention given their effects on image analysis results” [59]. Image artefacts interfering with MRI readings include radiofrequency (RF) intensity non-uniformity, phase-encode ghosting, signal wrap and geometric distortion due to gradient non-uniformity and B0 inhomogeneity.
Investigators of the Canadian MRI Consensus Group further specify that “a standardized MRI protocol is important during patient follow-up” [2]. The recommended brain MRI sequences are 3D FLAIR (or axial + sagittal FLAIR), post-gadolinium T1, axial T2 and/or PD, obtained with a minimum MRI field strength of 1.5 T and a slice thickness of 1 mm for T1 and ≤3 mm for FLAIR with no gap; total head coverage should include the entire brain and brainstem.
MSCare, the (US) Consortium of Multiple Sclerosis Centers [8], proposes a standardised MRI protocol that they regularly update (Table 15.2).
Table 15.2
MSCare guidelines for standardised MRI protocol
Standardized brain MRI protocol (diagnosis and routine follow-up of MS) | |
Field strength | Scans should be of good quality, with adequate signal-noise ratio (SNR) and resolution (in-sections, pixel resolution of ≤ 1mm × 1mm) |
Scan prescription Coverage Section thickness and gap | Use the subcallosal plane to prescribe or reformat axial oblique sections Whole brain coverage ≤3 mm, no gap (for 2D acquisition or 3D reconstruction) |
Core sequences | Anatomic 3D inversion recovery-prepared T1 gradient echo (e.g. 1.0–1.5 mm thickness) Gadolinium single dose 0.1 mmol/kg given over 30 secondsa 3D sagittal T2-weighted FLAIRb (e.g. 1.0 to 1.5 mm thickness) 3D T2-weightedb (e.g. 1.0 to 1.5 mm thickness) 2D axial DWI (≤5 mm slices, no gap) 3D FLASH (non IRc prep) post-gadoliniumb (e.g. 1.0 to 1.5 mm thickness) 3D series would be typically reconstructed to 3mm thickness for display and subsequent comparison for lesion counts |
Optional sequences | Axial proton attenuation Pre-or post-gadolinium axial T1 spin-echo (for chronic black holes) Susceptibility weighted imaging (SW1) for identification of central vein within T2 lesions |
These requirements are sufficient not only for visual assessment but also for automated image analysis software, since most packages performing MRI-based brain segmentation, atrophy computations or lesion segmentation work either on single MR images or on a subset of multi-parametric images, simultaneously taken into account.
15.4 Analysis Methods
15.4.1 Cross-Sectional Biomarkers
15.4.1.1 Brain Volume Computations
The volume of the whole brain, or volumes of brain structures, can be easily computed through brain segmentation techniques. Brain segmentation implies that the whole brain, its constituent tissue types or individual brain substructures can be identified based on MRI(s). A typical first step is “brain extraction” or “skull stripping”, a preprocessing step that ensures that only brain tissue is transmitted to the segmentation pathway. Various brain extraction methods, such as the brain extraction tool (BET) [55], brain surface extractor (BSE) [50], ROBEX [28], etc., are available. Approaches are diverse, including morphological, geometrical, image processing and modelling operations (hole filling, surface modelling, edge detection, intensity thresholding, atlas matching, deformable models, patch-based labelling, etc.). Moreover, the results of individual brain extraction methods can be enhanced by applying hybrid techniques, thus combining results from several individual methods.
After the first step of brain extraction, the process of brain segmentation can be started. This is typically based on a probabilistic modelling of voxel intensities, exploiting the fact that different tissue types have different MR image characteristics. Recent literature provides an excellent overview of brain segmentation methods [10]. Well-known and validated examples include FAST [61], SIENAX [56] and FreeSurfer [17]. Gaussian mixture models are popular; image intensities for each tissue type are modelled as a (sum of) Gaussian components. This modelling is usually performed using expectation–maximisation (EM), a well-known iterative parameter estimation algorithm. Spatial priors, serving as starting values and also as spatial constraints, can be obtained from appropriate brain atlases available in the literature [42]. The EM framework can be extended to intrinsically model some of the common distortions present in MR images, such as spatial inhomogeneity of image intensities known as bias field. Otherwise, such correction should be performed in preprocessing, e.g. using methods such as N3 or N4ITK [53, 58]. The EM results are probabilistic, i.e. each voxel is assigned probability of belonging to each of the classes of interest (WM, GM, CSF, etc.). These maps can be thresholded to obtain hard segmentations. Volumes in millilitres for each class can be computed either based on the hard or the fuzzy segmentation, by simply multiplying the sum of the tissue segmentation over all voxels by the voxel volume.
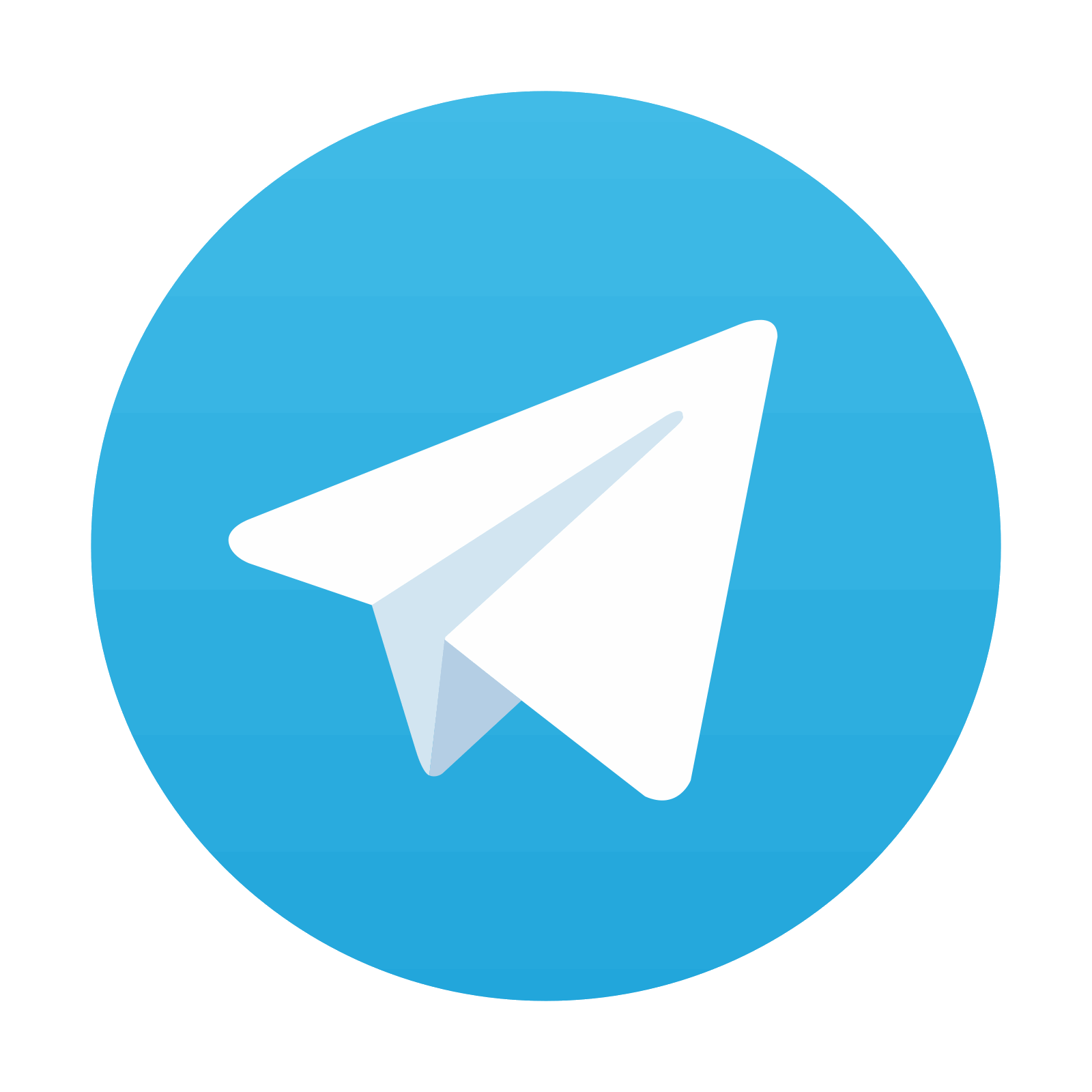
Stay updated, free articles. Join our Telegram channel
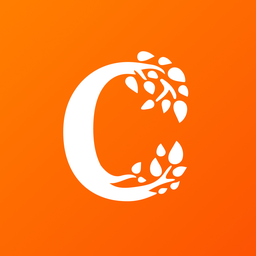
Full access? Get Clinical Tree
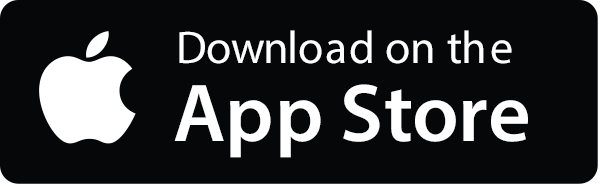
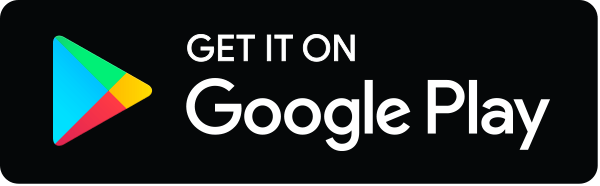