Fig. 8.1
Imaging LTP in the CA1 region. (A) A CCD image of the CA1 region in a hippocampal slice, with boundaries between layers highlighted in red. SO stratum oriens, SP stratum pyramidale, SR stratum radiatum, DG dentate gyrus. The CA2 region is above the field of view. The stimulating electrode is visible from the left (red arrow) and the field potential recording electrode is visible from the right (white arrow head). Four sites are indicated with numbers. (Bi). Optical traces are displayed from the 464 photodiodes that image the spatial distributions of responses to electrical stimulation (60 μA, 200 μs, each trace is an average of four trials). The red curves highlight boundaries in (A). (Bii). The field potential recording (above) and optical trace from the same location (site 1). The dashed trace represents the response 2 min before stimulation with three theta bursts and the red trace represents the response 50 min after. (Ci) The response map prior to theta burst stimulation, with amplitude encoded as color according to the scale in the lower left corner. (Cii) The response map after theta burst stimulation. (Ciii) The difference map created by subtracting (Ci) from (Cii) illustrates the spatial distribution of LTP. (D) The time course of response amplitude and field potential slope from the sites indicated in (A). Three theta bursts were applied at the arrow (TBS). (Modified from Chang and Jackson (2006b))
The basis for these features remains unclear, but the LTP maps bore a striking resemblance to the maps of action potential amplitude during the second and third theta burst used to induce LTP. Figure 8.2a displays the response amplitude map, along-side the spike amplitude maps during the three successive LTP-inducing theta bursts. There is a clear increase in spike map complexity with each theta burst, and the final spike map resembles the LTP map in the right panel. A plot of spike amplitude versus change in response amplitude shows a poor correlation for the first theta burst but a high correlation for the second two (Fig. 8.2b). The slope of these plots rose from ~0.35 to ~1 in the averages from eight such experiments (Fig. 8.2c). This experiment showed that regions where theta burst stimulation induced action potentials were subsequently found to be potentiated. This correlation reveals an important manifestation of the well-known Hebb condition of plasticity in which a stimulus can alter synaptic strength only when the stimulus drives action potentials in both the pre- and postsynaptic cells. Thus, this recasts the question of why LTP induction is so heterogeneous. The question has been translated into a question about why postsynaptic spiking is so heterogeneous. Although we are in no better position to answer this question than the first question, knowing that the action potential map exhibits the same heterogeneity suggests that experiments to study the action potential map may ultimately shed light on the basis for the spatial heterogeneity of LTP.
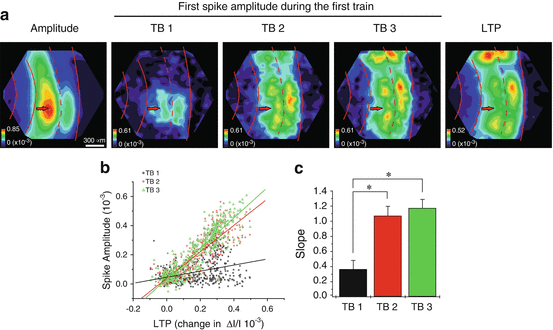
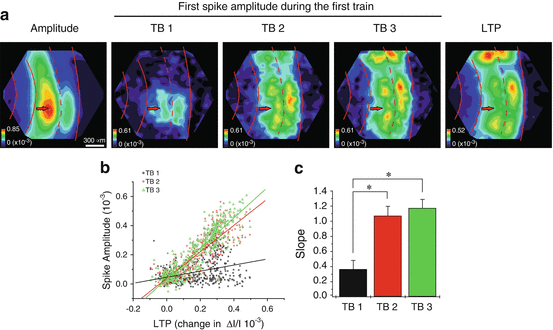
Fig. 8.2
Correlation between LTP and spike amplitude during induction. (a) Maps of response amplitude (first panel), spike amplitude during the first, second, and third theta bursts (next three panels), and LTP (right panel). Red curves highlight boundaries between layers as in Fig. 8.1a (Stimulation 80 μA). (b) Plot of spike amplitude during theta bursts versus the magnitude of LTP, with each point in the field of view contributing one point to the plots. The slopes increased with successive theta bursts. (c) The average slope from eight experiments as in (b) show statistically significant increases in slope after the first theta burst (*P < 0.005). (Modified from Chang and Jackson (2006b))
One consistent feature in LTP maps was that they usually display a minimum around the site of stimulation. This was seen in both the CA1 region (Chang and Jackson 2006b) and the dentate gyrus (Wright and Jackson 2014). In the CA1 region this feature did not simply reflect saturation of responses. It was seen with a range of stimulus currents. Another possible explanation for the gap is that local activation of more inhibitory interneurons by the stimulating electrode could cause greater local release of GABA. The extent of the minimum around the stimulating electrode would then reflect the extent of directly activated GABA release. This may explain the results in the CA1 region as these experiments were performed in the absence of GABAA receptor blockade. But in the dentate gyrus experiments a GABAA receptor antagonist was present (and was necessary to obtain LTP with this induction protocol), so local inhibition cannot account for this particular aspect of heterogeneity. Near the stimulating electrode neurons are depolarized both directly, and indirectly by the activation of synapses. The more global depolarization of the entire neuron could activate signaling pathways that counter the signaling pathways activated by synapses that lead to LTP.
Another interesting feature of the LTP map was that a peak consistently appeared at the edge of the CA1 region bordering the CA2 region. This site was also shown to be a hot spot for epileptiform activity (Chang et al. 2007). Interestingly, the narrow CA2 region separating this hot spot from the CA3 region has much less LTP and lower amplitude epileptiform activity. The CA2 region is a well recognized anatomical subfield of the hippocampus, but the adjacent CA1 border region had not been distinguished from the rest of the CA1 region previously. This appears to be an interesting functional specialization, yet to be explained in terms of structure or molecular composition. These distinctions in terms of plasticity may be relevant to the recent finding that the CA2 region plays a role in social memory (Hitti and Siegelbaum 2014).
3 Gating
LTP directly increases the amplitude of responses to the activation of a targeted synapse. But whether this increase ends at that target or propagates further will be very relevant to the potential for plasticity at a given class of synapses to influence behavior. This issue is especially important in circuits with dense intrinsic connections. LTP at intrinsic synapses, as opposed to projection synapses, have the potential to alter how activity travels through a brain region and on to the next station. In this way LTP can modulate a gate that determines what passes through a circuit. Considering the plasticity of a synapse in isolation from its context ignores a potential for rich and interesting consequences that LTP can have on circuit function through complex polysynaptic interactions.
The dentate gyrus has been proposed to serve as the gateway to the hippocampus, and serves as a critical station in filtering and processing the inputs the hippocampus receives from the entorhinal cortex through the perforant path (Ang et al. 2006; Dudek and Sutula 2007; Heinemann et al. 1992; Lothman et al. 1992). Dentate granule cells serve as the principle relays in this pathway and project with axons known as mossy fibers to the CA3 region. However, the dentate gyrus performs a great deal of processing of cortical inputs. Granule cells innervate nearby mossy cells, an excitatory neuron located in the hilus. Mossy cells are thought to play an important role in the processing of information by the dentate gyrus (Buckmaster and Schwartzkroin 1994; Henze and Buzsaki 2007; Scharfman and Myers 2013). Mossy cells receive inputs from granule cells and reciprocate with synapses on granule cells in a powerful pathway of recurrent excitation (Jackson and Scharfman 1996; Scharfman 1995; Scharfman et al. 1990). This circuitry is illustrated in Fig. 8.3. Applying stimulation to the outer molecular layer of the dentate gyrus activates the extrinsic inputs from the entorhinal cortex, and the granule cell-mossy cell circuit distributes activity broadly throughout the dentate gyrus.
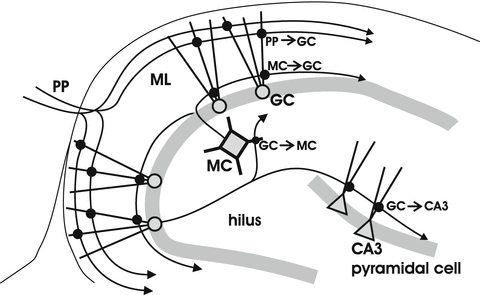
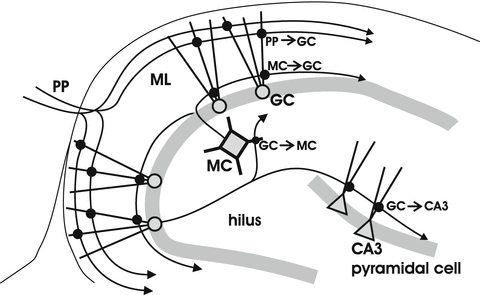
Fig. 8.3
Excitatory synapses of the dentate gyrus. The perforant path (PP) provides inputs from the entorhinal cortex to granule cell (GC) dendrites in the molecular layer (ML). GCs innervate mossy cells (MCs) in the hilus, which reciprocate with synapses on GCs. GCs also innervate pyramidal cells in the CA3 region (interneurons are not shown)
The phenomenon of LTP was first reported in the dentate gyrus of the rabbit at synapses between entorhinal cortex (perforant path) axons and granule cells (Bliss and Gardner-Medwin 1973). However, studies of the LTP of this pathway have yet to separate the contributions of cortical inputs from the mossy cell-granule cell circuit. This is especially difficult because the synapses are close together and the latencies are short. Synapses involving mossy cells do express LTP (Kleschevnikov and Routtenberg 2003; Lysetskiy et al. 2005) and strengthening these synapses would enhance responses to the activation of cortical inputs, thus creating the false impression of LTP at perforant path → granule cell synapses (Claiborne et al. 1993).
Voltage imaging has been used to evaluate responses and LTP throughout the dentate gyrus, and has provided some insight into the capacity of the different synapses for LTP as well as the impact of LTP at these synapses on the flow of activity through the dentate gyrus and on to the CA3 region. Applying theta bursts to the molecular layer potentiates responses throughout the molecular layer but does not potentiate responses in the CA3 region. However, applying theta bursts directly to granule cells potentiates responses in both the molecular layer and the CA3 region (Wright and Jackson 2014). The potentiation of molecular layer responses by granule cell theta bursts requires that LTP occur at either mossy cell → granule cell synapses, or granule cell → mossy cell synapses, or both (Fig. 8.3). Furthermore, it is intriguing that theta burst stimulation of inputs to granule cells in the molecular layer does not potentiate responses in the CA3 region but theta burst stimulation of granule cells does.
To identify the synapses potentiated by applying theta bursts to different sites, NMDA receptors were blocked with the NMDA receptor antagonist APV (Wright and Jackson 2014). NMDA receptor blockade should be very informative in this situation because the synapses formed by granule cells on CA3 pyramidal cells (mossy fiber synapses) express a form of LTP which is unusual in being resistant to NMDA receptor blockade. Thus, the block of LTP in the CA3 region induced by granule cell stimulation provides strong evidence against LTP of mossy fiber synapses. LTP of upstream synapses must be responsible for the robust potentiation in the CA3 region, and this implies LTP in dentate gyrus circuitry. It is further interesting that NMDA receptor blockade failed to inhibit LTP in the molecular layer induced by granule cell stimulation, but completely abolished LTP induced by theta burst stimulation of cortical inputs. Thus, synapses intrinsic to the dentate gyrus manifest an NMDA receptor independent form of LTP. This LTP most likely occurs in synapses formed by granule cell axons on mossy cells, as might be expected if NMDA receptor independent LTP is a general property of synapses formed by mossy cells.
Dual-site stimulation experiments (Fig. 8.4A1) provided an additional basis for evaluating the capacity of dentate gyrus synapses for LTP, and permitted unique tests of hypotheses that LTP at one set of synapses can enhance the response to other inputs. Thus, theta burst stimulation of cortical inputs potentiated responses to granule cell stimulation. This indicates that theta burst stimulation of cortical inputs does indeed induce LTP in downstream synapses between granule cells and mossy cells. Theta burst stimulation of granule cells potentiated responses in the molecular layer and CA3 regions (Fig. 8.4A3, B1, B2). Furthermore, this induction protocol not only potentiated responses to granule cells, but also potentiated the responses in the CA3 region to cortical inputs (Fig. 8.4A2, B1). This indicates that the mossy cell circuitry illustrated in Fig. 8.3 can amplify signals that enter the dentate gyrus through the perforant path from the cortex.
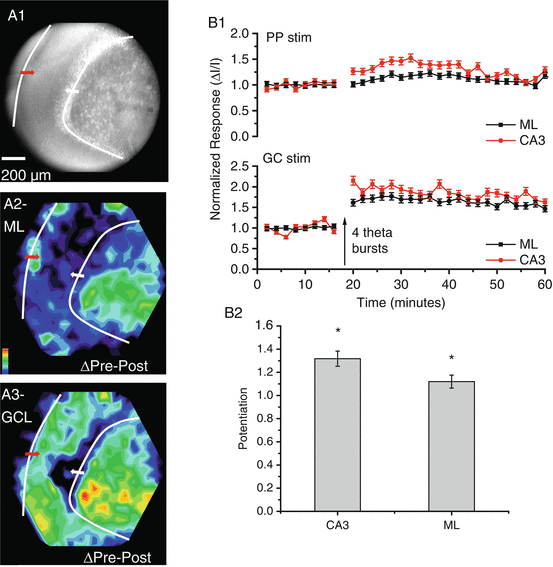
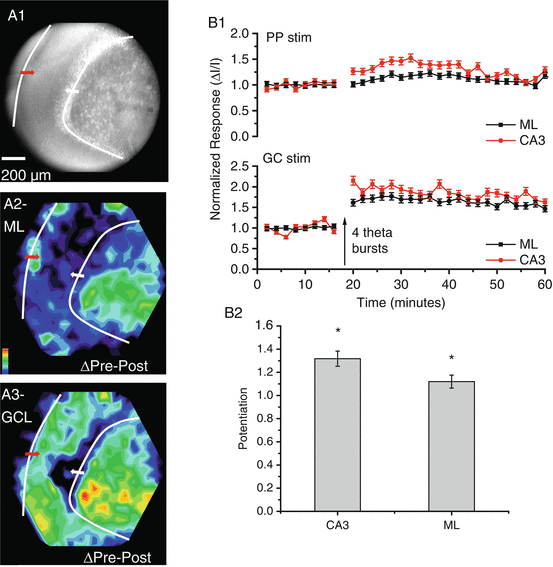
Fig. 8.4
Dual-site stimulation in the dentate gyrus. (A) A CCD image shows the dentate gyrus with stimulating electrodes positioned in the molecular layer to activate cortical inputs (red arrow) and in the granule cell layer to activate granule cells and MC inputs (white arrow). Four theta bursts were applied to the granule cell layer to induce LTP. (A2) The LTP map for responses to molecular layer stimulation shows that these responses were potentiated in the CA3 region. (A3) The LTP map for responses to granule cell layer stimulation shows that responses were potentiated in both the molecular layer and CA3 region. (B1) Time course plot of responses to stimulation of the molecular layer (PP, above) and GC layer (GC below). (B2) The heterosynaptic potentiation of responses to perforant path stimulation by theta burst stimulation of the granule cell layer. Responses in both the CA3 region and molecular layer were significantly potentiated (*P < 0.05)
The results of experiments with NMDA receptor blockade together with dual site stimulation in the dentate gyrus created an interesting conundrum. They clearly show that very strong potentiation of responses in the CA3 region can result from LTP in upstream synapses in the dentate gyrus rather than LTP of local mossy fiber synapses. However, LTP of only mossy cell → granule cell synapses, as occurs with NMDA receptor blockade, or only of granule cell → mossy cells synapses, as occurs with theta burst stimulation of cortical inputs, fails to potentiate responses in the CA3 region. This issue is difficult to resolve but one intriguing possibility is that enhancement of transmission to the CA3 region requires LTP of both granule cell → mossy cell synapses and mossy cell → granule cell synapses. This suggests that the mossy cell circuitry can gate the flow of activity through the dentate gyrus, but only when a special condition has been met. Only when LTP occurs in these two distinct populations of synapses will transmission to the CA3 region be enhanced. Indeed this could require LTP in reciprocally connected pairs of granule cells and mossy cells. Granule cells have a high threshold for spiking and this has been proposed to play an important role in filtering inputs and selecting patterns of activity for further processing and transmission to the CA3 region (Hsu 2007; Leutgeb and Leutgeb 2007). This novel gating condition of reciprocal LTP may play an important role in how experience modifies the processing of information by the dentate gyrus, and alters the flow of information to the CA3 region.
4 Spatial Patterns and the Storage of Information
The nervous system takes in information from the environment and transforms it into patterns of activity distributed through neural networks, encoding the information as specific sets of activated neurons. If a pattern of activity leaves an imprint upon the network then some of the information will be stored. If the imprint enables a subsequent input to reactivate the original pattern, then the prior sensory input can be recalled. These ideas form the basis for many theories of information storage and recall by the nervous system, and in these theories LTP generally serves as the imprinting mechanism (Gerstner and Abbott 1997; McNaughton and Morris 1987; Rolls and Treves 1994; de Almeida et al. 2007; Rolls 2013).
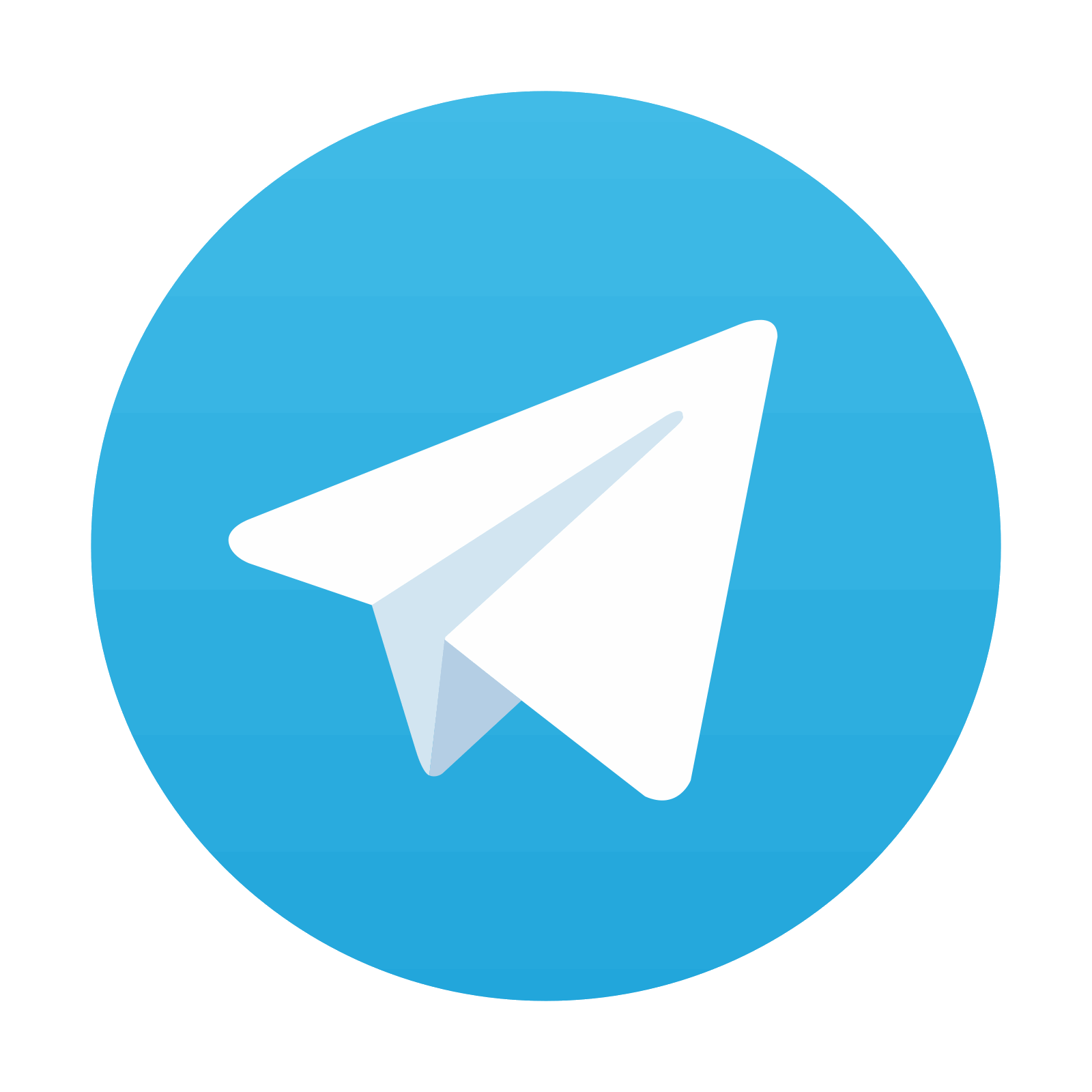
Stay updated, free articles. Join our Telegram channel
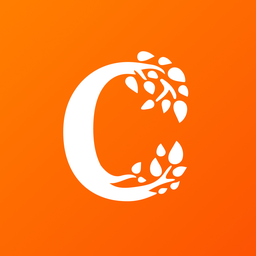
Full access? Get Clinical Tree
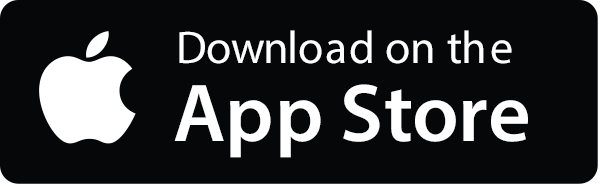
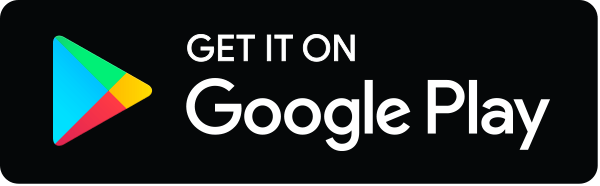