Fig. 5.1
99mTC-HMPAO brain death scintigraphy in a 16-year-old female with cardiac arrest and clinically brain death. There was no evidence of brain perfusion on perfusion images (a). No cerebral and cerebellar activities were also detected on delayed anterior (b) and lateral (c) images
Single-photon emission tomography (SPECT) acquisition is more useful to evaluate cerebellar activity or in cases of skull hyperemia or a photopenic area in the skull resulting from hematoma or a hardware. If planar view is used exclusively, at least one anterior and two lateral views are required, to test, respectively, for cerebral hemisphere flow and cerebellar hemisphere activity. It is also advised that the images be reviewed on the monitor with different color scales and different intensities. The final report should include information pertaining to the radiotracer, the dose, the technique, and the extent and severity of decreased flow. According to the SNM guideline, in the case of the absence of flow to the brain, the study should be interpreted as providing “no evidence of brain perfusion,” rather than “demonstrating brain death” [12].
Brain death scintigraphy is a reliable and accurate study. In a study by Facoo et al., SPECT imaging confirmed the diagnosis of brain death in 95.7 %, i.e., 45 out of 47 patients (aged 10 days to 75 years). In the other two clinically brain-dead children (aged 10 days and 12 months, respectively), weak perfusion of the basal ganglia, thalamus, and/or brain stem was still present, precluding the diagnosis of brain death; both of them died a few days later [22].
Brain activity may be observed, in certain cases, especially in infants, despite the clinical diagnosis of brain death [23–25]. Two patterns of brain death have been described by Palmer et al. [26]. The first one, which is more common, is associated with increased intracranial pressure. If the increased intracranial pressure is compensated by a mechanism or decreased in time by reduced edema, there is a risk of a false-negative (falsely normal) study. This may occur in infants with open fontanels and flexible skulls. Posttraumatic fractures, as well as the presence of a ventriculoperitoneal shunt, may represent other causes of reduced intracranial pressure [27, 28]. In the second scenario, nonfunctioning brain cells are not associated with increased intracranial pressure. While it is not common, a diagnostic imaging modality which evaluates only the flow to the brain may falsely be interpreted as a negative study. Further investigation is required to confirm whether radiotracers which show brain function such as 99mTc-HMPAO and 99mTc-ECD are more accurate in these scenarios. In a study by Ashwal and Schneider, brain activity was reported in 6 of 17 infants with clinical brain death, three of which were preterm infants [29]. Okuyaz et al. also reported that two newborns needed a second image to confirm the diagnosis [30]. In this study, eight patients who fulfilled the clinical criteria for brain death were evaluated using 99mTc-HMPAO SPECT; six patients demonstrated lack of flow activity in the cerebrum in their first SPECT study. If there is low perfusion to the brain or clinical deterioration, it may be helpful to repeat the study [17].
In summary, brain scintigraphy is a simple, noninvasive, reliable, and accurate ancillary test to demonstrate whether brain perfusion is present in patients that have been clinically diagnosed with brain death. Brain-specific radiotracers such as 99mTc-HMPAO and 99mTc-ECD are usually preferred. However, flow images and delayed acquisition, as well as SPECT study (in certain cases), are essential for the diagnosis. Cerebral blood flow may be detected in infants who are clinically brain dead, and repeating the study may be useful, in order to demonstrate the absence of perfusion and confirm the diagnosis of brain death.
5.3 Pulmonary Emboli
Within nuclear medicine studies performed in the acute care setting, pulmonary emboli (PE) represent the most common indication for the evaluation of the pulmonary system. PE is less common in the pediatric population than in adults. However, with improved chances of survival in chronically ill pediatric patients, the risk of complications such as PE has also increased [31]. Several studies show that PE is underdiagnosed in pediatric patients; physicians usually do not ask for the necessary examinations to evaluate for PE [31–34]. The clinical findings of PE in children may be subtle and variable and may mimic other diseases [31]. Shortness of breath, pleuritic chest pain, and hemoptysis are more common in children. In adolescents, pleuritic chest pain is the most common presenting symptom, followed by dyspnea, cough, and hemoptysis [35, 36]. Other symptoms include wheezing, crackles, sweating, tachycardia, nausea, vomiting, syncope, murmur, cyanosis, and pleurisy with friction rub [35, 36].
Many risk factors have been suggested as causing a predisposition in children to thromboembolism [36]. In a study conducted in a tertiary referral hospital by Biss et al., immobility, central venous line, and recent surgery represented the most common risk factors [33]. Cancer, vascular malformations, nephritic syndrome, long-term total parenteral nutrition (TPN) administration, systemic lupus erythematosus, ventriculoatrial shunts, congenital or acquired thrombotic tendencies, and acute deep venous thrombosis are the other risk factors associated with PE in children [10, 35]. Unlike adults, clinical prediction algorithms based on clinical signs, symptoms, and risk factors have not yet been validated in children [35, 36].
Arterial blood oxygen, erythrocyte sedimentation rate, and the electrocardiogram have yielded variable results for patients with PE. Chest x-ray is often normal in pediatric patients with PE. Atelectasis, pleural effusion, elevated hemidiaphragm, infiltrates, and consolidation are nonspecific findings that may be seen on the chest x-ray [35]. Oligemia distal to PE (Westermark sign) and a shallow, wedged-shaped opacity with its base against the pleura (Hampton hump) are rarely seen [10, 35]. Conventional pulmonary angiography, long considered the gold standard for the evaluation of PE, is infrequently used because of the availability of noninvasive imaging modalities with high sensitivity and specificity [37]. There are limited studies available to confirm the value of magnetic resonance (MR) angiography for the evaluation of PE. However, MR angiography may play a significant role in future.
Ventilation/perfusion scan (V/Q scan) and pulmonary computerized tomography angiography (CTA) are noninvasive studies which, depending on availability and local protocols, are usually used in order to test for PE in adults. With CTA’s recent increase in availability, and the high sensitivity and specificity of the study (which is usually greater than 95 %), pulmonary CTA is now the most common procedure for the diagnosis of PE [38]. Pulmonary emboli present as filling defects in the pulmonary arteries on CTA. CTA can be obtained quickly in critically ill patients.
CTA presents some disadvantages, mainly a high radiation exposure dose and the use of iodinated contrast. The effective radiation dose from V/Q scan is 1.2–2 mSv [39]. Depending on the technique, the effective radiation dose with CTA is between 5.4 and 19.9 mSv [39–41]. If CTA is not available, a V/Q scan may be considered, especially in order to reduce the radiation dose or if the patient has an allergy to iodinated contrast material or renal insufficiency [36, 42]. In a study by Stein et al., the use of an imaging algorithm with chest radiography, V/Q scan, and CTA for patients with a clinical suspicion of PE was found to reduce radiation exposure by 20 %, from 8.0 to 6.4 mSv, with no significant difference in the false-negative results. When the chest radiograph is normal, Stein et al. suggest performing a V/Q and acquiring CTA if there is an abnormality detected by the chest x-ray [43].
5.3.1 Radiopharmaceuticals and Imaging Technique
5.3.1.1 Perfusion Agent
Currently, 99mTc-macroaggregated albumin (99mTc-MAA) is the radiopharmaceutical used for lung perfusion imaging. In order to be trapped in the precapillary arterioles in the first pass, the size of a particle should be greater than the size of a capillary, which is approximately 7–10 μm. The particle size with 99mTc-MAA ranges from 10 to 90 μm (90 % between 10 and 90 μm; 60–80 % between 10 and 30 μm) and should not exceed 150 μm [44]. After slow injection into a peripheral vein in a supine position, 99mTc-MAA particles are trapped in precapillary arterioles, causing a temporary occlusion in a distribution proportional to regional arterial blood flow in the lungs. If the size of the particles is too large, the distribution may not exactly reflect the lung perfusion, as some of the particles may trap in the more proximal arterioles. The particles are then cleared by enzymatic hydrolysis and are eventually phagocytized by the reticuloendothelial system, with a biological half-life of 6–8 h in the lungs.
In general, the number of particles ranges from 150,000 to 500,000, which theoretically obstructs approximately 0.1 % of the total arterioles [45]. However, in certain clinical conditions, involving, for instance, pulmonary hypertension and right-to-left shunt, and in the pediatric population, the number of particles should be reduced in order to avoid any hemodynamically significant compromise in pulmonary circulation. In children, the number of particles should be adjusted for age or weight, so that the number of particles may be kept as low as reasonably possible. This is in order to avoid obstruction of more than 0.1 % of the total lung capillaries [45].
In neonates, the number of pulmonary capillaries is about 10 % that of adults. By the age of three, this number increases to 50 % of the adult number and reaches the adult number between the ages of 8–12 years old. Thus, the number of injected particles should not exceed 50,000 in a newborn (usually 10,000–30,000), 165,000 for a 1-year-old, and 200,000–300,000 for children between the ages of 5 and 10 (with an average weight of 20–35 kg) [45–47]. According to the North American Consensus Guidelines, if 99mTc is used for the ventilation study, the recommended injection doses are 2.59 MBq/kg (0.07 mCi/kg) and 1.11 MBq/kg (0.03 mCi/kg), with a minimum dose of 14.8 MBq (0.4 mCi), if no concomitant 99mTc ventilation study is performed [44, 48].
It is suggested that the patient lie down in a supine position for a few minutes before injection. A direct injection is preferred (rather than into an intravenous tube), and care should be taken not to withdraw blood back into the syringe, to avoid the formation of small clots which may present as hot spots on the perfusion images. If 99mTc-labeled radioaerosols or Technegas are used for the ventilation scan, then the ventilation scan should be performed first. If 81mKr gas is used, the ventilation scan can be conducted before or after the perfusion scan, or both examinations can be acquired simultaneously [44, 45].
Image acquisition can be obtained using a high-resolution, low-energy or a low-energy all-purpose (LEAP) collimator. Planar images are acquired in multiple projections (8 views are preferable) in a 128_128 or 256_256 matrix format, with 300,000–500,000 counts per image. Both lungs should be in the field of view. For cameras with small crystals (<300 mm in diameter), a diverging collimator is preferable [44]. A SPECT or SPECT/CT study may also be performed. The CT portion is acquired with a low-dose CT for attenuation correction and anatomic correlation [44, 45]. Several reports support that SPECT improves the performance of a pulmonary perfusion study [49–52]. In the majority of those studies, the sensitivity and specificity of the SPECT perfusion scan, in the diagnosis of PE, were at least 90 % [49–52].
5.3.1.2 Ventilation Agents
99mTc-DTPA Aerosol
99mTc-DTPA aerosol is the most commonly used radiopharmaceutical. 99mTc sulfur colloid aerosol may also be used, but 99mTc-DTPA is preferred in children because of the fast renal clearance [45]. The advantage of aerosols is the ability to obtain multiple views or a SPECT study, since radioaerosols do not wash out rapidly like radioactive gases. 99mTc-DTPA aerosol can be inhaled through a mask connected to a nebulizer containing a saline solution of the radiopharmaceutical. In older children, the use of a mouth piece and nose clips is preferable [10]. The ideal aerosol particle size is between 0.1 and 0.5 μm. Larger particles tend to deposit in the central airways (central deposition) which may degrade the images and also lead to inadequate radiotracer delivery to the alveoli. The patient is encouraged to rinse her mouth during and after the inhalation phase and avoid swallowing, since the activity in the saliva may cause high gastrointestinal activity in the esophagus and stomach. This may lead to a difficulty in visualizing the left lower lung field [45]. The aerosol particles cross the alveolar-capillary membrane and clear via the kidneys with a half-life of approximately 60 min in adults [53]. This clearance may increase in certain conditions, such as during exercise, and decrease with certain lung diseases such as cystic fibrosis [54].
The calculated administered dose is approximately 30 mCi (1110 MBq) in adults, adjusted in children according to body surface area and weight. The patient should inhale enough aerosol to deposit 37 MBq (1 mCi) radiotracer in the lungs (with a minimum activity of 10 MBq in children), to allow for sufficient count statistics [45]. When both perfusion and ventilation studies are performed with 99mTc labeled agents, the second study should have at least three times more count rates. Thus, it is usually suggested that the radioaerosol ventilation study be obtained before the perfusion scan.
133Xenon
133Xenon is an inert gas with a half-life of 5.24 days and energy of 81 KeV. After inhalation, 133xenon equilibrates across the alveolocapillary membrane and distributes throughout the body. The clearance of 133xenon from the body is very rapid, with a biological half-life of approximately 30 s [43]. The usual dose for children is 10–12 MBq/kg (0.3 mCi/kg), with a minimum dose of 100–120 MBq. Because of its low-energy photopeak, the ventilation study with 133xenon should be obtained before the perfusion scan with 99mTc-MAA, in order to avoid downscatter from 99mTc (this may degrade the 133xenon ventilation images). Since the activity is rapidly washed out, a posterior projection is generally used, with the study being acquired in three phases: an initial single breath-hold phase, followed by the equilibrium and washout phase [45].
To begin the study, a closed system must be set up, with a mouthpiece connected to the spirometer system. The patient is then asked to take a deep breath and hold it for 5–10 s or until an image of about 100,000 counts is obtained. This is difficult for some patients to tolerate, especially pediatric patients, or when the patient is critically ill or tachypneic. In the second equilibrium phase, after the initial breath, the patient is asked to breathe a mixture of air and 133xenon at the tidal volume rate. Usually two images are acquired, lasting 90 s each. In the third phase, the patient breathes the room air and exhales into a trapping system. During the third phase, three or four 45 s images are usually acquired. Since some of the 133xenon may escape during exhalation and enter the room air, it is necessary, for radiation safety procedure, to maintain a negative pressure in the room with an exhaust vent. This is usually placed near the floor and serves to remove the heavy atoms of 133xenon leakage.
99mTc-Technegas
Technegas is the other form of aerosol. It is generated by evaporating pertechnetate at a very high temperature in a graphite crucible in the presence of argon gas. This produces ultrafine particles of 99mTc-carbon, which acts like a gas. The majority of particles are between 30 and 60 nm. Technegas is inhaled via a facemask in a well-ventilated room. The inhalation may cause transient hypoxemia; this can be overcome by giving oxygen via nasal cannula [45]. Because of the smaller particle size, there is less central deposition in Technegas than in 99mTc-DTPA aerosols, especially in patients with obstructive airway diseases.
81mKrypton
81mKrypton gas is produced by a rubidium generator (81Rb/81mKr) with a very short half-life of 13 s and emissions of a 190 KeV gamma ray. Since the half-life is very short, only the wash-in images are feasible during inhalation. The short half-life is advantageous because of radiation protection (there is no need for a special exhaust system) and the low radiation dose to the lungs. The other advantage is that it can be continuously administered, and the images can be obtained in multiple projections. There is also a minimal need for patient cooperation, which is advantageous in cases involving critically ill patients or children.
Because of the higher energy and short half-life of 81mkrypton, the images can be taken alternatively with 99mTc-MAA perfusion acquisition in each projection, without moving the patient in between. There is no downscatter from 81mkrypton (190 KeV) energy to 99mTc-MAA (140KeV), because there is no significant activity from 81mkrypton at the time of perfusion imaging. However, the generator is expensive, and since 81Rb has a half-life of 4.7 h, it can only be used for one working day [44, 45].
5.3.2 Interpretation
The activity should be homogeneous in both perfusion and ventilation scans (Fig. 5.2). An uncomplicated pulmonary embolus usually presents as a perfusion defect in a region with normal ventilation (the so-called “mismatched” perfusion defect) (Fig. 5.3). However, other possibilities may mimic this pattern. On the other hand, a matched defect may be also due to an embolus resulting from infarction or to emboli superimposed on underlying parenchymal diseases. Therefore, in addition to the presence or absence of perfusion and ventilation defects, other parameters such as the number, size (small, moderate, or large), and pattern (nonsegmental versus segmental) of perfusion and ventilation abnormalities are helpful for appropriate diagnosis.
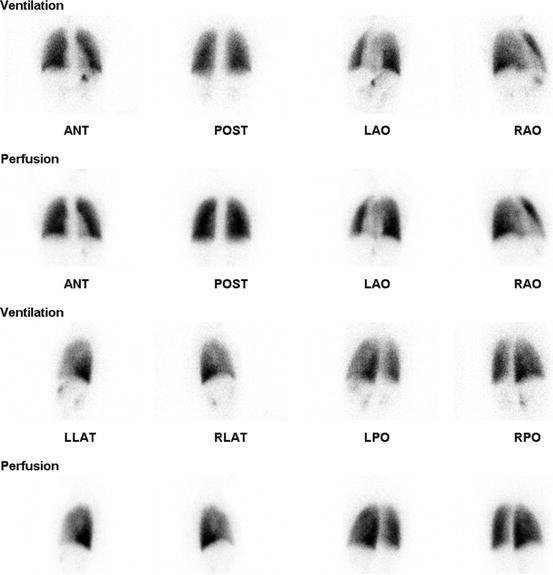
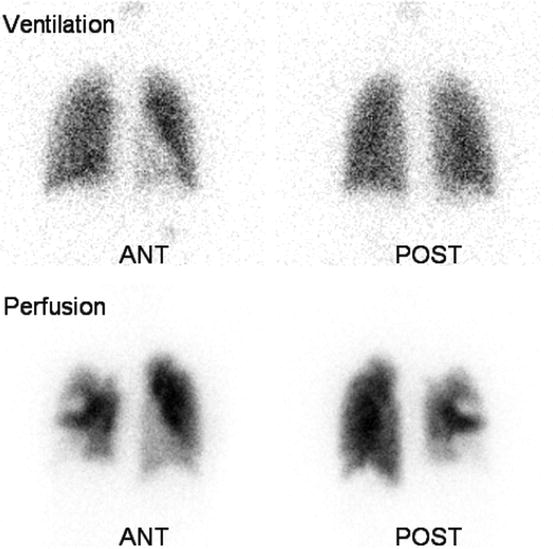
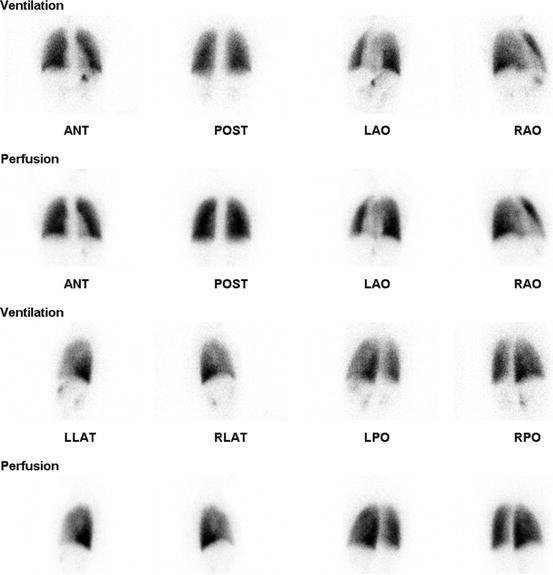
Fig. 5.2
15-year-old female with radiofrequency ablation in the right atrium admitted at hospital for tachypnea. There was no segmental or nonsegmental perfusion and ventilation defect to suggest PE
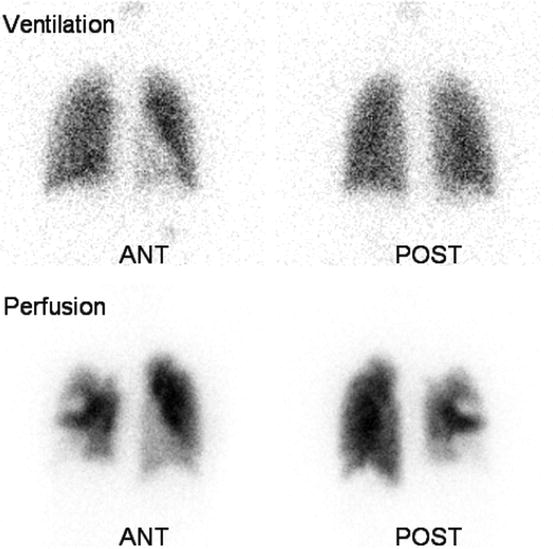
Fig. 5.3
16-year-old female with deep vein thrombosis and tachypnea. On lung ventilation/perfusion scan, multiple mismatched perfusion/ventilation defects are noted in both lungs (more on the right), suggestive of high probability of pulmonary emboli. Only the anterior and posterior views are shown here
Many diagnostic schemes have been introduced to aid in the interpretation of the V/Q scan, including Biello, Prospective Investigation of Pulmonary Embolism Diagnosis (PIOPED) and modified PIOPED (II) criteria. These criteria usually define the likelihood of pulmonary emboli as low, intermediate, or high. However, these criteria have not been validated in children and are not fully described in this chapter. In practice, physicians do not usually follow strict criteria, but rather use different criteria and their own experience (“Gestalt” interpretation) [10, 55].
Regardless of the diagnostic algorithms, the likelihood of PE is very low (<5 %) in a normal perfusion scan. The probability of pulmonary emboli is also low when the perfusion defects are small (<25 % of a segment), matched on the ventilation scan, or associated with a larger abnormality on the chest radiography. The probability of pulmonary emboli is high when there are two or more perfusion defects (at least one should be moderate or large in size) that are not accompanied by a ventilation or chest x-ray abnormality. The interpretation of a single moderate-sized segmental V/Q mismatch is controversial and challenging. The risk of PE is variable with this finding, so it is reclassified into the intermediate probability in modified PIOPED criteria similar to Biello criteria [56]. Stein et al. demonstrated that if there is no underlying cardiopulmonary disease, a single moderate-sized segmental V/Q mismatch has a positive predictive value (PPV) of up to 86 % [57]. Thus, it can be put in the high probability category.
In practice, it is preferable to have less intermediate or indeterminate results. Glaser et al. proposed a trinary interpretation, in which the normal, low, and very low probabilities are interpreted as reflecting no evidence of PE, a high probability as reflecting the presence of PE, and an intermediate probability as nondiagnostic [58]. In this study, the single segmental V/Q mismatch was categorized as a positive PE. Glaser et al. also compared their new scheme with the traditional probability algorithm and found similar outcomes with no significant difference in the rates of false-negative results (P = 0.63). According to the trinary interpretation, 8.4 % of the examinations were interpreted as PE positive, 3.5 % as nondiagnostic, and 88.1 % as PE negative (the total number of examination studies was 664). In a pediatric subgroup analysis of 20 children, the examinations were positive in 10 %, nondiagnostic in 5 %, and negative in 85 %, with no false negatives using either scheme. They concluded that the trinary interpretation scheme can be safely applied to children.
In a study by Gelfand et al. of children with no known lung disease or previous extensive PE, it was found that the use of the Biello criteria with minimal modification yielded a low rate of indeterminate results (15 %) [59]. Gelfand et al. point out that the V/Q scan involves less radiation than CTA and can be safely used in children who are suspected of having PE. In our institution, the percentage of indeterminate/intermediate studies is about 10 % (unpublished). With the use of a SPECT or SPECT/CT for both ventilation and perfusion scans, the diagnostic accuracy of the V/Q scan will be even greater, and the rate of indeterminate studies will decrease [60, 61]. However, the value of SPECT or SPECT/CT for evaluating PE has not been validated in children.
In summary, the V/Q lung scan is a simple and safe examination method that entails less radiation exposure than CTA and can be used to test for PE in children. The indeterminate results in children and adolescents are low in the selected group with no known history of lung disease. Therefore, it is still appropriate to perform a V/Q lung scan in children in the evaluation of PE and in a hemodynamically stable patient with no history of lung disease and normal chest radiograph.
5.4 Hepatobiliary Scintigraphy
Hepatobiliary scintigraphy is a noninvasive modality with high sensitivity and specificity for the diagnosis of acute cholecystitis. It may also be useful for evaluating bile leakage post liver and gallbladder operation. The hepatobiliary scan is also helpful in the identification of biliary patency in infants with suspicion for biliary atresia.
Although cholecystitis is less common in children than in adults, its incidence in children is believed to be increasing [10]. It is more common in adolescent girls than adolescent boys. Acute cholecystitis in adults is usually associated with the obstruction of the cystic duct, secondary to cholelithiasis. Accumulated mucus causes increased pressure and gallbladder distension, edema, and bile stasis, leading to bacterial overgrowth and inflammation. Acute cholecystitis secondary to gallstones is infrequent in children but may be seen with hemolytic anemia [62]. Acalculous cholecystitis in children is usually associated with other disease conditions such as sepsis, infections (e.g., streptococcal infections), vasculitis (e.g., Kawasaki’s disease), trauma, sickle cell disease, malignancy, and metabolic and congenital diseases. It may also associate with prolonged total parenteral nutrition (TPN) or occur following surgery [62–64]. In acute acalculous cholecystitis, partial or complete obstruction of the cystic duct occurs secondary to the formation of biliary sludge in the absence of gallstone. The associated inflammation and edema may compromise blood flow and lead to bacterial infection [62, 65].
Children usually present with abdominal pain in the right upper quadrant or epigastric region; this is sometimes accompanied by nausea, vomiting, anorexia, and fever. On physical examination, right upper quadrant tenderness is the most common finding [10]. Other findings may include tachycardia, palpable enlarged gallbladder, and jaundice. The absence of physical findings does not exclude the diagnosis of acute cholecystitis. Leukocytosis is a common laboratory finding [65]. Alanine aminotransferase (ALT) and aspartate aminotransferase (AST), bilirubin, and alkaline phosphatase levels may be elevated in cholecystitis. Laboratory tests may be needed to exclude other causes of abdominal pain (e.g., amylase/lipase for pancreatitis, urinalysis for pyelonephritis and renal calculi, and pregnancy tests if the patient is of childbearing age).
Although the sensitivity and specificity of ultrasound (US) are usually less than that of the hepatobiliary scan, US is considered the primary imaging modality in patients with suspicion of acute cholecystitis. This is mainly because of its availability, the ease with which it is performed (at the patient’s bedside), the lack of ionizing radiation, and the ability to evaluate for other causes of acute abdominal pain [66]. Using US, a large gallbladder with a thickened wall and surrounding fluid accumulation, stones, and local tenderness over the gallbladder (radiologic Murphy sign) may be detected. The sensitivity and specificity of US are estimated to be in the range of 40–97 % and 64–100 %, respectively, in adults [37, 67–69]. In children, the sensitivity of the ultrasound in the detection of cholecystitis is probably lower, likely because cholecystitis tends to be more chronic than acute [70].
CT scan is not conducted as an initial imaging test for the evaluation of cholecystitis but can be obtained for the evaluation of extrabiliary disorders and complications of acute cholecystitis. CT scan’s diagnostic findings include the presence of gallstones, a thickened gallbladder wall, pericholecystic fluid collections, subserosal edema, gallbladder distention, and sludge [71]. However, the sensitivity of CT scan is less than that of the US. MRI has also been performed in acute cholecystitis in pregnant women, with indeterminate US findings for eliminating radiation exposure.
Hepatobiliary scintigraphy has the highest diagnostic accuracy among the diagnostic imaging modalities to evaluate for acute cholecystitis [66]. In a systemic review by Kiewiet et al. examining the accuracy of different imaging modalities in patients with suspected acute cholecystitis, the sensitivity and specificity of hepatobiliary scintigraphy were 96 and 90 %, respectively [66]. The sensitivity of hepatobiliary scintigraphy was significantly higher than that of ultrasound (81 %) and MR imaging (85 %). However, in practice, hepatobiliary scintigraphy is usually reserved for patients with indeterminate findings on US or a negative US but a high suspicion of acute cholecystitis.
Hepatobiliary scintigraphy can be useful in differentiating between biliary atresia and other causes of jaundice in infants. The clinical, biochemical, and sometimes histologic findings may be similar in neonatal hepatitis and biliary atresia [72]. Early diagnosis of biliary atresia is important, since the outcome is most successful with early surgery, while surgery is not indicated in patients with neonatal hepatitis [72, 73]. In a study by Gerhold et al., the sensitivity and specificity of hepatobiliary scintigraphy in the diagnosis of biliary atresia were 97 % and 82 %, respectively [74].
Hepatobiliary scintigraphy is also useful in evaluating for a biliary leak after trauma or surgery (both open surgery and laparoscopic surgery on the biliary system and gallbladder). The clinical presentation varies widely, depending on the type of injury and the amount of leakage. US is usually considered the initial test to investigate the presence of leakage after surgery. Fluid collection is US’ main finding [75]. Biliary dilatation is often absent, since the biliary system is decompressed by the leak. Bile leakage may be also detected on CT scan. In general, both US and CT scan cannot detect active leakage, and usually, there is a need for follow-up US studies to confirm the diagnosis. Hepatobiliary scan is an accurate modality for the detection of active bile leakage with high contrast and lack of interference from adjacent structures or bowel gas [72, 76].
5.4.1 Radiopharmaceuticals and Imaging Technique
99mTc-disofenin (DISIDA) and 99mTc-mebrofenin (BrIDA) are the most common radiotracers used for hepatobiliary scintigraphy [77]. These radiotracers are injected intravenously and are rapidly taken up by the hepatocytes and excreted into the biliary system. The administered activity for infants and children is 1.85 MBq/kg (0.05 mCi/kg), with a minimum administered activity of 18.5 MBq (0.5 mCi). In infants with hyperbilirubinemia, mebrofenin is the preferred agent, and in 24 h, delayed images are sometimes required; the minimum administered activity is 37 MBq (1.0 mCi).
The patient should be fasting for 4 h prior to the test. However, fasting is not required for the evaluation of biliary atresia in infants. For the evaluation of biliary atresia, premedication with phenobarbital (5 mg/kg/day) for 3–5 days before hepatobiliary scintigraphy increases bile secretion and the specificity of the test [77]. Ursodeoxycholic acid (20 mg/kg every 12 h for 48–72 h) has also been also used in the pretreatment regimen to stimulate bile secretion [77].
Following IV injection of the radiotracer, dynamic images are obtained in supine position with a gamma camera equipped with high-resolution, parallel-hole collimator viewing the entire abdomen, including the liver. The study is recorded with serial 1.0 min frames for 60 min using a 128 × 128 matrix with zooming, based on the patient size. Additional sequential static images are usually obtained based on the indication and initial findings on the first 60 min images. For evaluation of acute cholecystitis, if the gallbladder fails to be visualized in the first 60 min study, the image acquisition should be extended for up to 4 h after the radiotracer injection or 30–60 min after morphine sulfate (0.04 mg/kg intravenously over 2–3 min) augmentation [77]. Contraindications to the use of morphine include increased intracranial pressure in children (absolute), respiratory depression in nonventilated patients (absolute), morphine allergy (absolute), and acute pancreatitis (relative) [77]. When there is no or questionable bowel excretion, 24 h images are sometimes required for the evaluation of biliary atresia.
If the gallbladder does not empty significantly during the initial 60 min period, the images will repeat after a standard fatty meal or cholecystokinin analog (sincalide; 0.02 μg/kg diluted with saline to 30 ml with a maximum dose of 1.4 μg/70 kg infused for 30–45 min). Sincalide may also be used when the patient is fasting for more than 24 h, to empty the gallbladder in order to avoid a false-positive study.
For the evaluation of a biliary leak, additional images in various projections and in different timings (usually delayed images) are obtained in order to identify any abnormal collection of the radiotracer.
5.4.2 Interpretation
If the gallbladder is not visualized by 60 min, the image acquisition will continue for an additional 3–4 h or for 30–60 min after the morphine augmentation. During this period, nonvisualization of the gallbladder on a hepatobiliary scintigraphy is suggestive of acute cholecystitis (Fig. 5.4). However, in children, visualization of the gallbladder does not completely exclude cholecystitis, as gallbladder visualization, though infrequent, is possible in acalculous cholecystitis or in the partial obstruction of the cystic duct [62]. If the gallbladder is not visualized in the first 60 min, but is seen on additional images after 60 min, the diagnosis of chronic cholecystitis is suggested. Morphine augmentation at 60 min may be helpful to reduce the time of images and the number of false-positive studies (gallbladder nonvisualization in the absence of acute cholecystitis). However, in acute acalculous cholecystitis or partial obstruction of the cystic duct (which is more common in pediatric patients), it may overcome functional obstruction of the cystic duct and result in a false-negative examination [62].
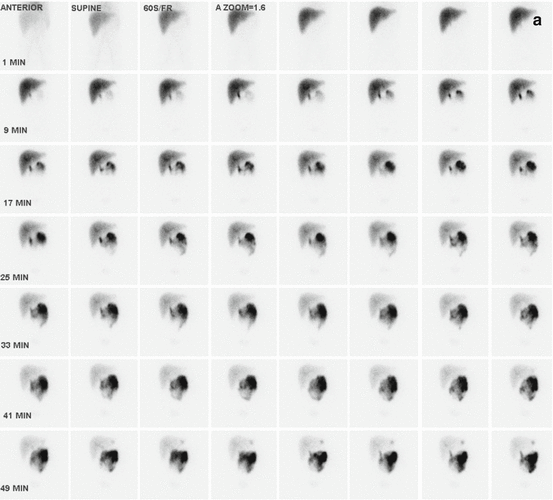
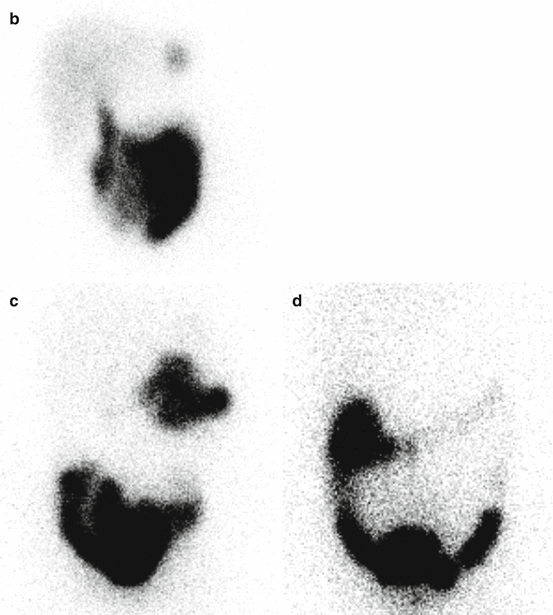
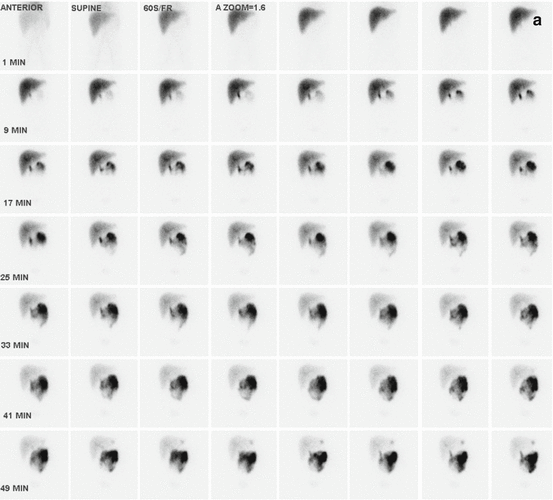
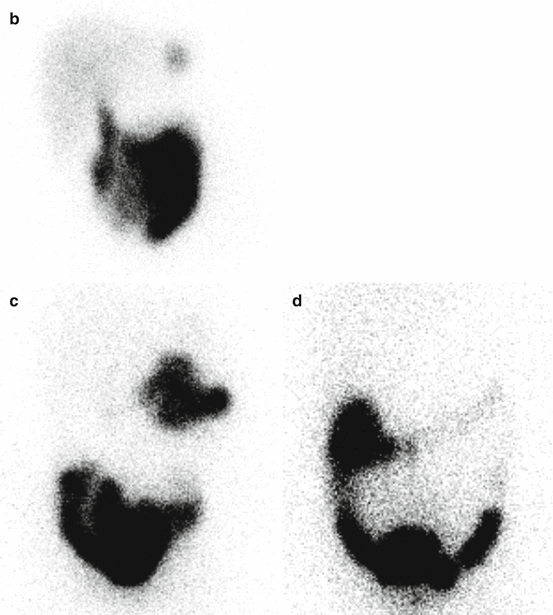
Fig. 5.4
Hepatobiliary scan in a 5-year-old girl with abdominal pain. Ultrasound showed a thick-wall gallbladder. On hepatobiliary scan, there is good extraction and excretion of the radiotracer by hepatocytes (a). The activity is visualized in the bowel at about 9 min with no evidence of intrahepatic or extrahepatic biliary duct dilatation. Gallbladder is not visualized even on delayed images [at 60 (b), 120 (c), and 240 (d) minutes]. Of note, at about 50 min, a focus of activity is identified in the left upper abdomen likely due to the reflux of the radiotracer into the stomach. The study is suggestive of acute cholecystitis or obstruction in the cystic duct
The causes of a false-positive study (gallbladder nonvisualization in the absence of acute cholecystitis) include inadequate or prolonged fasting (less than 2 h or more than 24 h), severe hepatocellular disease, high-grade common bile duct obstruction, severe concomitant illness, rapid biliary-to-bowel transit (insufficient tracer activity remaining in the liver for delayed imaging), severe chronic cholecystitis, and previous cholecystectomy [77]. The causes of a false-negative study (gallbladder visualization in the presence of acute cholecystitis) include visualization of a bowel loop simulating the gallbladder, acute acalculous cholecystitis, the presence of the dilated-cystic-duct sign simulating the gallbladder, bile leak due to gallbladder perforation, and congenital anomalies simulating the gallbladder [77].
Hepatobiliary scintigraphy may exclude the diagnosis of biliary atresia by detecting the biliary drainage of the radiotracer into the bowel (Fig. 5.5). If liver uptake is normal and no bowel activity is identified within 24 h, a diagnosis of biliary atresia is suggested (Fig. 5.6) [78]. However, nonvisualization of the bowel activity within a 24 h period following the radiotracer injection may be seen in other conditions, including severe cases of neonatal hepatitis, Alagille syndrome, dehydration, sepsis, TPN cholestasis, and bile plug syndrome in cystic fibrosis. In neonatal hepatitis, the hepatocyte uptake is reduced and the hepatobiliary transit time into the bowel is delayed [62]. If the hepatocyte uptake is relatively preserved but the transit to the bowel is delayed (i.e., the bowel activity is ultimately visualized), the study is suggestive of intrahepatic cholestasis [74].
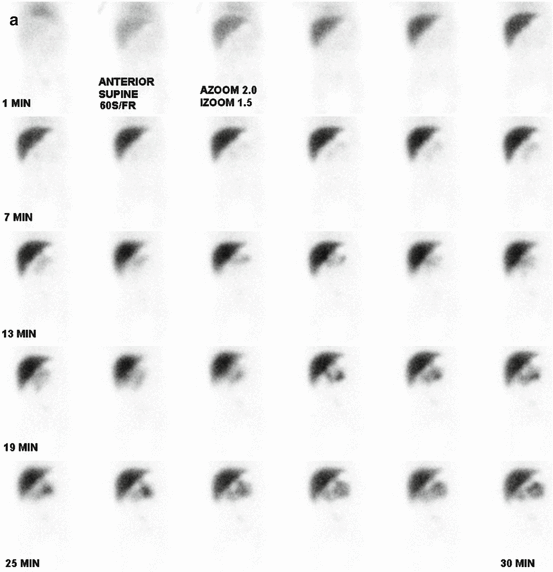
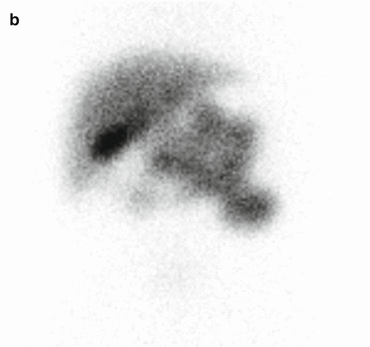
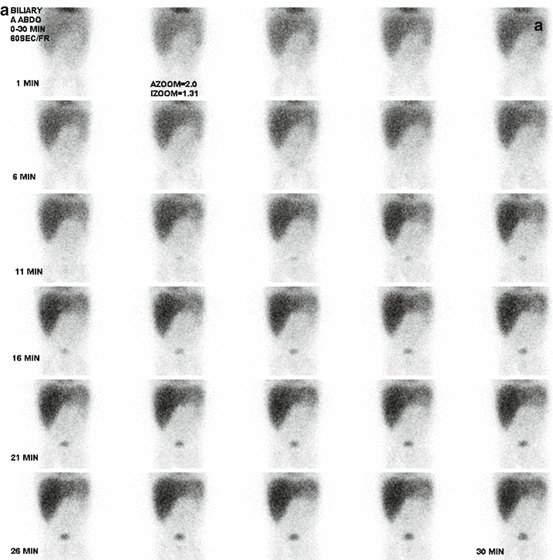
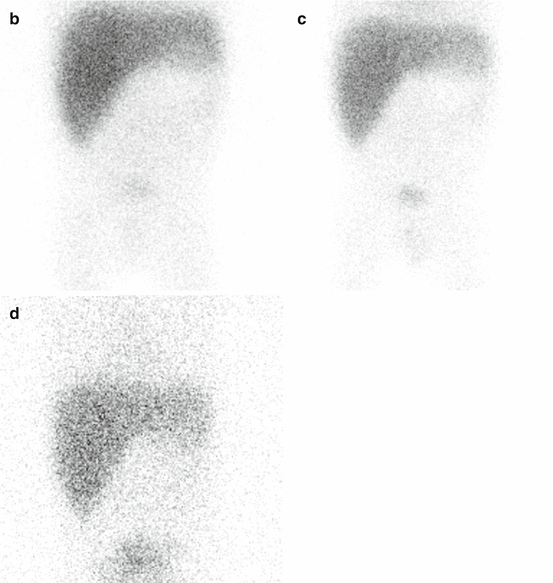
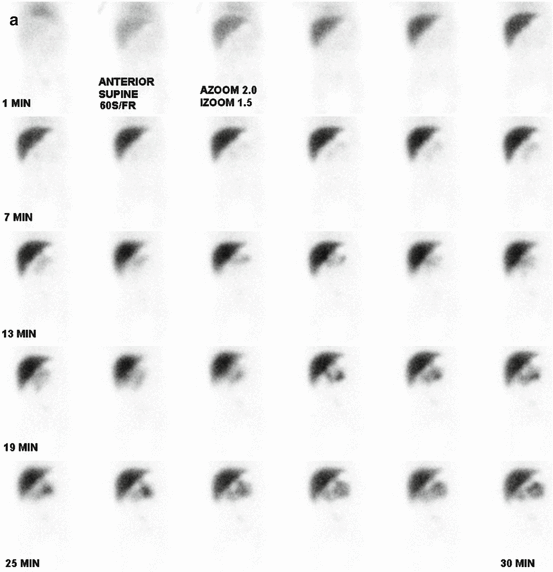
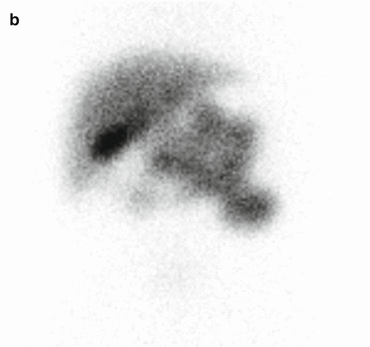
Fig. 5.5
Hepatobiliary study in a 3-month-old boy with conjugated hyperbilirubinemia. There are normal extraction and excretion of the radiotracer by hepatocytes with good drainage into the bowel (a). Gallbladder is visualized at 60 min (b) and drained later (not shown here). The study excludes the possibility of biliary atresia
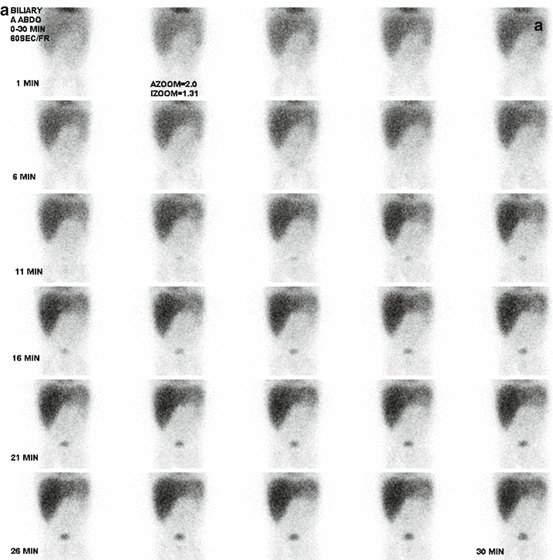
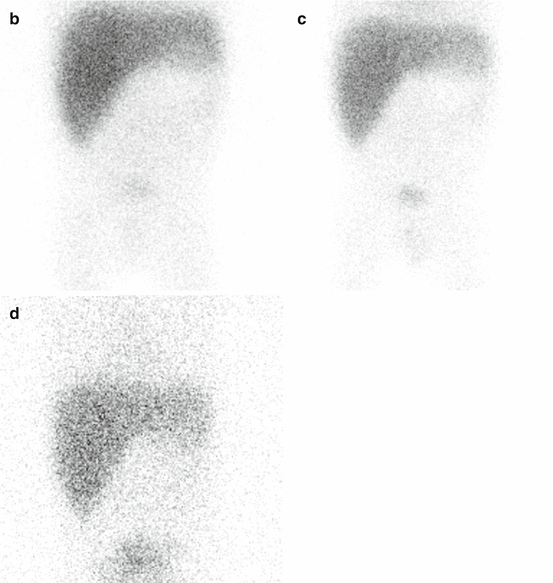
Fig. 5.6
Four-month-old girl with jaundice. On hepatobiliary scan, there was good extraction of the radiotracer by hepatocytes (a). However, no activity was seen in the bowel at 60 (b) and 240 min (c) and even on delayed images at 24 h (d)
Hepatobiliary scintigraphy is also useful when the presence of a bile leak is suspected in patients with acute abdominal pain, especially after liver operation or cholecystectomy [79]. Multiple sequential images in multiple projections, including delayed views, particularly in the right paracolic gutter, are often necessary to confirm the presence of bile leakage [37]. Knowledge of the previous operation and correlation with anatomical imaging (e.g., CT scan) are useful for accurate diagnosis. A SPECT/CT may be useful in cases with equivocal findings on planar images [80].
5.5 Lower GI Bleeding
Lower gastrointestinal (GI) bleeding is relatively common in infants and children. Although in the majority of cases it is benign and self-limited, in some cases, it is severe and warrants prompt management. The approach is to stabilize the patient and then to correct the underlying diseases. There are numerous underlying possibilities including coagulopathies, Meckel diverticulum, intussusceptions, infectious enterocolitis, intestinal duplication, malrotation with volvulus, polyps, GI allergy, and inflammatory bowel disease. Accurate localization and detection of the underlying pathology is important for therapy management. Endoscopy and angiography are commonly used to detect the site of bleeding and to identify the underlying causes. However, in many occasions, bleeding is intermittent and the result of the anatomical modalities is negative. 99mTc-labeled RBC scintigraphy is the most sensitive imaging modality for detecting active bleeding, is noninvasive, and can depict both arterial and venous sources of bleeding [81]. Meckel diverticulum is one of the common causes of painless GI bleeding in infants and young children. Meckel diverticulum scintigraphy is a simple, noninvasive method for the evaluation of Meckel diverticulum containing ectopic gastric mucosa.
5.6 Meckel Scan
Meckel diverticulum (MD) is a true diverticulum and the most common congenital anomaly of the GI tract [82]. This anomaly is a remnant of the omphalomesenteric duct. Patients are usually asymptomatic. Ectopic gastric mucosa is present in approximately 50–60 % of the symptomatic cases and in more than 95 % of those patients who present with bleeding [83, 84]. The complications include pain, bleeding and/or perforation (from the secretion of ectopic gastric mucosa), and intussusceptions [83–85]. Although the complications may occur at any age, more than 50 % of patients with complications, mainly painless bleeding, are seen before 2 years of age [86, 83].
Different imaging modalities, including barium study, ultrasound, angiography, and CT scan, have been introduced to detect MD [87]. 99mTc-pertechnetate is the most common and accurate noninvasive method to investigate for MD containing ectopic gastric mucosa with overall sensitivity of 85 % and specificity of 95 % in children.
5.6.1 Radiopharmaceuticals and Imaging Technique
Meckel scintigraphy is performed after the intravenous injection of 99mTc-pertechnetate. The administered activity is 1.85 MBq/kg (0.05 mCi/kg) with a minimum dose of 9.25 MBq (0.25 mCi) and maximum dose of 370 MBq (10 mCi) [48]. Patient preparation is important to perform an optimum examination. The patient is recommended to be fasting for 4 h. However, in emergency situation, the study can be done without fasting [88]. It is important to determine if the patient had another study before the examination to avoid any possible interference with Meckel scintigraphy. Previous use of perchlorate can suppress the gastric mucosa and should be avoided [78, 89]. Residual barium from a prior study may result in a false-negative examination. Previous study with in vivo RBC labeling may interfere with the study since the injected 99mTc-pertechnetate will bind to the circulating RBCs which were treated with stannous ion via intravenous administration of a cold pyrophosphate kit. This is not a problem with in vitro RBC labeling scan [88].
Certain premedications may increase the sensitivity of the test. However, they are not considered necessary for the study, especially in emergency situation. Histamine H2-receptor antagonists, such as cimetidine, ranitidine, and famotidine, inhibit the secretion of pertechnetate into the gastric mucosa without affecting the uptake. The recommended dose for cimetidine is 10–20 mg/kg/day for neonates and 20 mg/kg/day for infants and older children, orally for 2 days before the study, or 300 mg in 100 mL of 5 % dextrose intravenously over 20 min, one hour before the radiotracer injection [88]. The recommended dose for ranitidine is 1 mg/kg given intravenously (maximum 50 mg) over 20 min, 1 h before the test [88]. For famotidine, it is recommended to administer 0.25 mg/kg intravenously, 1 h before the scanning [88]. Pentagastrin (with a dose of 6 μg/kg subcutaneously) can increase the gastric uptake [88]. Glucagon relaxes the smooth muscles and decreases the peristalsis of the GI tract and, therefore, decreases the washout of the tracer. However, it decreases tracer uptake in the gastric mucosa and should be used in combination with pentagastrin. The recommended dose for glucagon is 50 mg/kg intravenously, to a maximum of 1 mg, diluted to a volume of 10 mL with sterile water, infused slowly over two minutes immediately before radiotracer injection. Glucagon should not be administered to diabetic patients [88].
Imaging usually starts immediately after the radiotracer injection, in supine position, using high-resolution collimator. A radionuclide angiogram is acquired at intervals of 1–5 s for 1 min to assess for vascular malformation and possibly to detect the site of bleeding. This is followed by a continuous imaging (1 min/frame) or sequential static images every 3–5 min, for 45–60 min in anterior projection. Delayed static images are sometimes needed to evaluate equivocal findings or to exclude other causes of focal activity in the abdomen and pelvis, such as urinary activity. Lateral images can be helpful to differentiate an MD (usually is located anteriorly) from the urinary activity (which is normally located posteriorly). Routine post-void imaging is recommended to assess MD located adjacent to or behind the bladder. Bladder lavage, nasogastric suctioning, repeating the study in equivocal cases, and SPECT or SPECT/CT are other techniques that have been reported to increase the sensitivity [88–90].
5.6.2 Interpretation
MD typically presents as a focus of increased activity in the abdomen or pelvis, most frequently in the right lower quadrant (Fig. 5.7). The activity is usually visualized a few minutes after radiotracer injection, increasing over time simultaneously with the gastric uptake. The intensity may change over time due to intestinal secretions, hemorrhage, or increased motility of the bowel. Lateral views (to differentiate MD from ureter and bladder), upright position images (to identify fixed organs such as the duodenum), and delayed images are helpful for accurate diagnosis. Meckel scan has 90 % accuracy, 85 % sensitivity, and 95 % specificity to detect the ectopic gastric tissue in children [85]. The sensitivity and specificity are much lower in adults. A number of conditions and pathologies may also present with focal increased activity and cause false-positive results (Table 5.1). Although they are categorized as false positive for MD, some of them are the real pathology and need treatment. These include duplication cysts or enteric duplication containing ectopic gastric mucosa, intestinal obstruction, intussusception, inflammation, ulcers, and vascular tumors. False-negative studies may be seen with a poor technique, low uptake of gastric mucosa in infants, smaller MD, infarction or impaired blood supply to the MD, and residual barium from the previous study (Table 5.1).
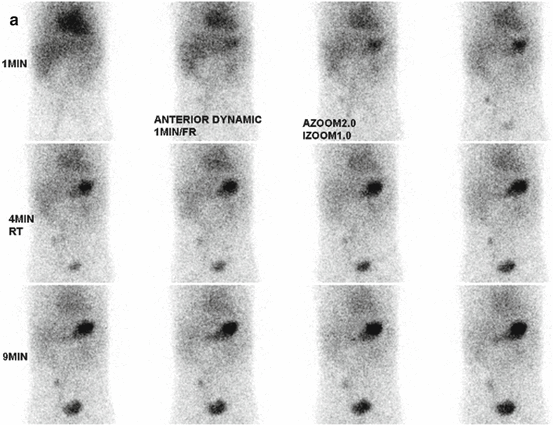
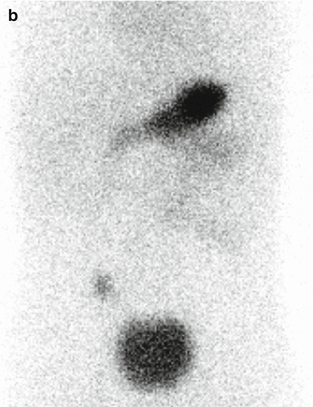
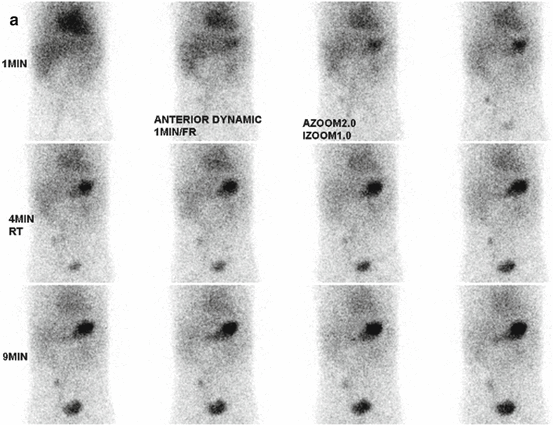
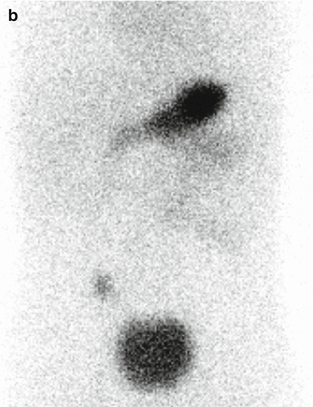
Fig. 5.7
(a) Meckel scan in a 2-year-old boy with painless bleeding. A focal activity is visualized in the right lower quadrant simultaneously with the gastric uptake. (b) Delayed static image showed persistent focal uptake in the right lower quadrant. The diagnosis of Meckel diverticulum with heterotopic gastric and pancreatic tissues was confirmed on pathology
Table 5.1
Possible causes of false-negative and false-positive results in a Meckel scintigraphy
False negative | Mechanism | Examples |
Lack of sufficient gastric mucosa | Absent or insufficient gastric mucosa | |
Washout of the secreted 99mTc-pertechnetate | Dilution of radioactivity as a result of hemorrhage Fast washout because of increased bowel movement | |
Impaired vascular supply | Intussusception Obstruction Pressure from the adjacent dilated ureter, bladder, or bowel | |
Poor technique | Inadequate preparation (e.g., no fasting, lack of pharmacologic intervention, prior administration of potassium perchlorate, prior barium study, aluminum hydroxide ingestion) Imaging technique (inadequate timing of imaging, lateral and postvoid images to evaluate any possible activity behind hot organs) Insufficient radioisotope | |
False positive | Urinary system | Ureter, dilated pelvis, extrarenal pelvis, hydronephrosis, bladder diverticulum, vesicoureteral reflux, ectopic kidney |
Vascular | Aneurysm of vessels, angiodysplasia, hemangioma, arteriovenous shunt | |
Hyperemia | Inflammation (e.g., inflammatory bowel disease, gastritis, regional enteritis, colitis, juvenile colon polyps, appendicitis, abscess, small bowel obstruction, surgery, laparoscopy, or endoscopy) Neoplasm (e.g., colon cancer, carcinoid, lymphoma, uterine fibroid, leiomyosarcoma) | |
Other sites of ectopic gastric mucosa | Duplication cyst, Barrett’s esophagus, ectopic gastric mucosa in other sites of gastrointestinal tracer including thr pancreas, colon, and duodenum | |
Others | Contamination (usually from urine) Physiologic activity (e.g., salivary or gastric secretion) |
5.7 99mTc-RBC Scan
Both 99mTC-sulfur colloid (99mTc-SC) and 99m Tc-red blood cell (99mTc-RBC) can be used for the assessment of GI bleeding. 99mTc-RBC is generally accepted as the radiopharmaceutical of choice for evaluating lower GI bleeding [62]. The advantages of using 99mTc-RBCs is that it remains intravascular allowing a longer imaging time over the course of several hours maximizing the chance of detecting intermittent bleeding [72, 91]. Using 99mTc-RBCs scintigraphy, bleeding rates as low as 0.1–0.4 mL/min may be detected; the lowest volume of bleeding to be detected by angiography is 0.5 mL/min [10, 81, 92].
Alternatively, 99mTc-SC can also be used to assess lower GI bleeding. 99mTc-SC has a high bleeding to background activity ratio which allows detecting rates as low as 0.05–0.1 mL/min. However, imaging can be acquired only for 30–45 min which limits the possibility to detect intermittent bleeding. In addition, the high tracer accumulation in the liver and spleen may obscure adjacent bleeding activity sites [10].
Before the examination, the clinical situation of the patient should be assessed to make sure that the patient is stable or should be monitored by a physician or nurse. History of abdominal surgery, any previous medication (may be the cause of GI bleeding or may interfere with RBC labeling), and other diagnostic and therapeutic studies should be reviewed [92].
5.7.1 Radiopharmaceuticals and Imaging Technique
There are three methods to label the RBCs: in vitro, modified in vivo, and in vivo. The labeling efficiency is highest with the in vitro labeling (>95 %) [93]. The disadvantage of lower labeling efficiency using in vivo method is the possible secretion of free pertechnetate within the gastrointestinal tracer and/or excretion of free pertechnetate into the urinary system which may lead to false-positive results [62]. The recommended dose is calculated based on the European Association of Nuclear Medicine (EANM) pediatric dosage card, which uses a baseline dose of 56 MBq (1.51 mCi) multiplied by a weight-based multiple [92, 94]. The resulting minimum administered activity is 80 MBq (2.16 mCi) for a 3 kg child and the maximum administered activity is 784 MBq (21.19 mCi) for a 68 kg patient [92, 94].
After injection of the radiotracer, flow study is acquired for 60 s (1 s/frame) followed by dynamic imaging for 60–120 min in supine position. It is recommended not to obtain serial intermittent static images, and the recommended frame rate should not exceed one frame per 60 s [92]. Additional delayed static images in different projections can be obtained as needed. In order to detect intermittent bleeding, the patient can be imaged continuously for as long as it is practical to detect the bleeding source [92]. If no bleeding is detected, delayed images up to 24 h may be helpful to identify any intermittent bleeding.
5.7.2 Interpretation
GI bleeding is seen on 99mTc-RBC scan as activity outside the expected anatomic blood pool structures, which increases progressively over time, with antegrade or retrograde motion of tracer along the bowel lumen [92]. The movement of activity is best detected on cinematic display and may occur very rapidly. Accurate identification of the location of bleeding requires knowledge of the anatomy and reviewing the cinematic display. Small bowel bleeding may be distinguished from a colonic source by its rapid curvilinear movement through a series of multiple, small, centrally located segments on cinematic display of the abdomen. In contrast, in the large bowel bleeding, there is a linear elongated pattern of activity with peripheral location within the abdomen [62, 92]. If the extravasated activity is visualized only on delayed images (e.g., 24 h), the location cannot be accurately identified as the bleeding may originate elsewhere in the GI tract with interim antegrade and retrograde movement [95–97]. Although some authors argued the value of delayed images in detection of the site of bleeding, others suggested that it may have some benefits in terms of patient management in order to decide for blood transfusion, surgery, or angiography [92].
There are a number of findings that may be misinterpreted as the site of bleeding (false positive), including but not limited to free 99mTc-pertechnetate (secondary to swallowed salivary gland activity or from excreted gastric mucosa activity), blood pool activity in other organs or hypervascular lesions (penile activity, variable uterine activity, uterine leiomyoma), urinary activity (the activity in the kidneys, ureter, bladder diverticulum, pelvic kidney), vascular abnormalities (e.g., aortic aneurism), liver hemangioma, splenosis, and inflammatory bowel disease [92]. Reviewing lateral views and cinematic display, and correlation with anatomical modalities are usually helpful to distinguish these findings with GI bleeding. The majority of these false-positive findings present as a fixed activity with no antegrade or retrograde movement. The study will be positive when the patient has an active bleed. If the image acquisition is done very late in the patient management, the chance of a negative radionuclide study will be higher. Other pitfalls include misinterpretation because of infrequent image acquisition or incorrect localization based on single delayed images [98].
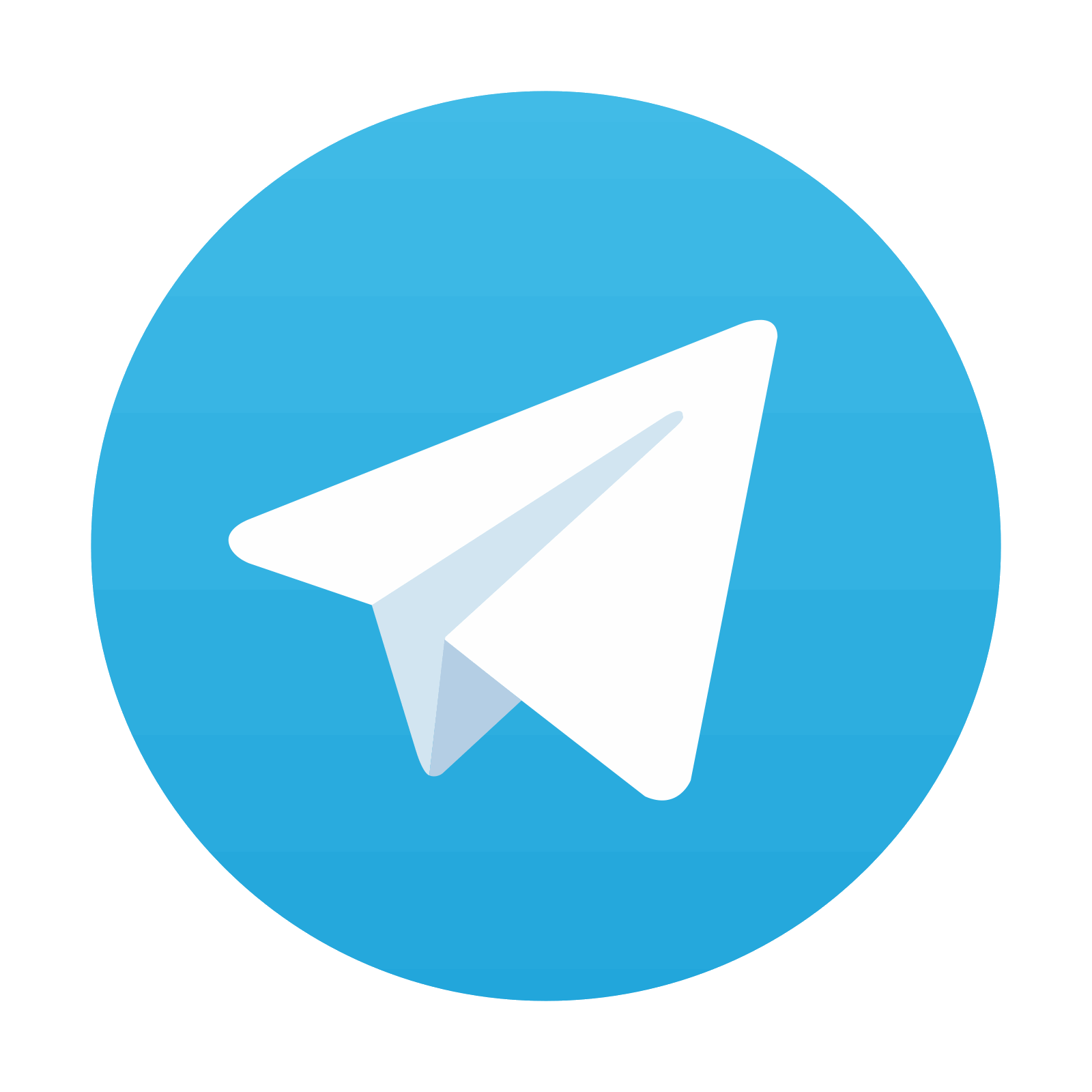
Stay updated, free articles. Join our Telegram channel
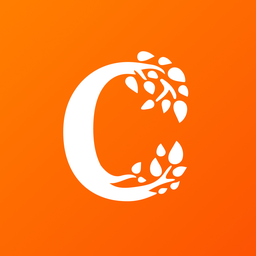
Full access? Get Clinical Tree
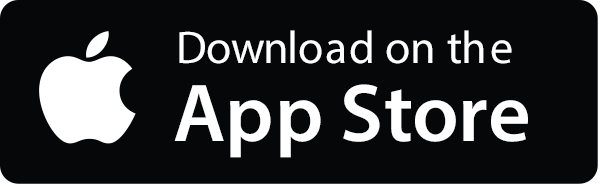
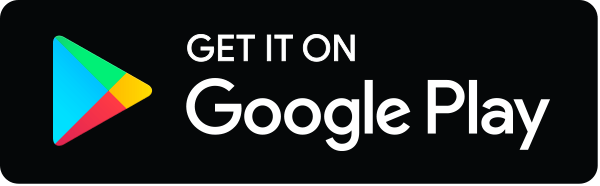