Fig. 23.1
The evolution of MR images over time. In 1974, the first image of a small animal was obtained by Lauterbur using a technique called zeugmatography at that time. The Mansfield’s group obtained the first human anatomical image in vivo, an axial cross section of a finger (1977). On 28 August 1980 in Aberdeen, the first clinical image of a cross section of the abdomen was obtained. In the late 1980s, the use of 1.5 T magnetic resonance began to spread around the world followed in the late 1990s by 3 T systems. In the 21st century the MR image acquired at 7 Thas an in-plane resolution of 192 μm in plane
The trend forward high magnetic fields derives from the quite low sensitivity of MRI. This originates from the small difference in the population of two consecutive Zeeman energy levels caused by the difference in energy of these levels. The slight excess of spin in the fundamental state generates a small polarisation, which depends on the temperature and the magnetic field applied, and it is only from this small fraction of sample that the whole absorption signal is detected in correspondence with the radio frequency associated with the nuclear transition. One direct method for increasing the low sensitivity was identified as increasing the static magnetic field B0. In more detail, the MR signal increases in quadratic mode with the strength of the static magnetic field, while the associated noise demonstrates linear dependence. Therefore, the use of MR 7 T equipment allows a significant increase in the signal-to-noise ratio (SNR) in comparison with lower fields. For example, an image obtained with a clinical 1.5 T or even a 3 T system has a spatial resolution limit of about 1 cubic millimetre, while the combination of the benefits of ultra-high field can lead to a resolution of some hundredths of microns with similar signal-to-noise ratio. The significant increase in SNR produces a great improvement of all the imaging parameters and can be used not only in terms of spatial resolution but also in terms of sensitivity to modifications of the composition of the tissue or in terms of temporal resolution for dynamic phenomena or in terms of spectral resolution of signals. In addition, many other benefits could come out from the potential of new sources of mechanisms of contrast. Particularly in the brain, but also generally in the human body, there are potential sources of signal which cannot be fully explored using standard magnetic fields, because of low SNR, poor spatial resolution and/or their scarcity. It is the case of myelin, iron or metabolites which contain nuclei that differ from hydrogen (13C, 23Na, 31P) that can be in principle detected by MR. These further signal sources can be extremely useful for providing additional and complementary information about molecular structure and/or the physiological, metabolic and functional dynamics of physiopathological processes. Moreover, the improvement in SNR and consequently in the intrinsic sensitivity of MR experiment can make possible the study of spontaneous distribution of nuclei different from proton that are normally found within structures of biological interest. For the same reason, it becomes possible to explore the distribution and the metabolic dynamics of molecular probes that are artificially enriched with stable isotopes that are visible in MR.
Increasing the magnetic field however causes some physical and instrumental problems that must be considered and fully investigated so that the potential of an ultra-high field MR system can be fully exploited. The major issues are related to the signal losses associated with the effects of magnetic susceptibility and inhomogeneities of the static magnetic field B0, to the increase of chemical shift artefacts and to the variations in the relaxation times T1 and T2, which completely change the semeiotics of the images and the strategies of signal acquisition.
Moreover, the wavelength and dielectric effects of the radio frequency (RF) signal produce an uneven excitation and can cause an inhomogeneous distribution of radio frequency energy deposition on tissues. At 7 T, the resonance frequency for hydrogen is 298 MHz. Raising the operating frequency leads to the so-called wavelength effect: the radio frequency wavelength becomes comparable with the dimensions of the sample being investigated (limbs, brain and trunk) which per se brings stationary wavelength effects to the whole sample. The experiment becomes more complicated due to the dielectric properties of the sample irradiated by the radio frequency that further decreases the radiation wavelength (e.g. at 7 T, the wavelength in anatomical tissue with a high concentration of water is about 12 cm). This effect shows up as inconsistencies in the RF field transmitted (B1) distribution, which is known as dielectric resonance. This generates peculiar artefacts in images that present hypo- and hyperintense zones caused by the presence of peaks and dips in the RF magnetic field B1 and as a consequence generates locally different flip angles. The inhomogeneities in the B1 magnetic field inevitably translate into inhomogeneous depositing of energy on the patient and give rise to possible “hot spots” of energy deposited. Specific absorption rate (SAR) is the parameter that measures this energy and forms the basis for both national and international patient safety standards. Commercial UHF MR systems monitor this parameter and use acquisition thresholds to do not exceed the above-mentioned standards. However, given the uneven distribution of the transmitted fields and the possible presence of “hot spots”, new methods for assessing variations in local magnetic field are mandatory. These may involve the simulation of electromagnetic fields and experimental measures [1], as well as the development of sensors for the real-time monitoring of SAR specifically for each individual patient (Fig. 23.2).
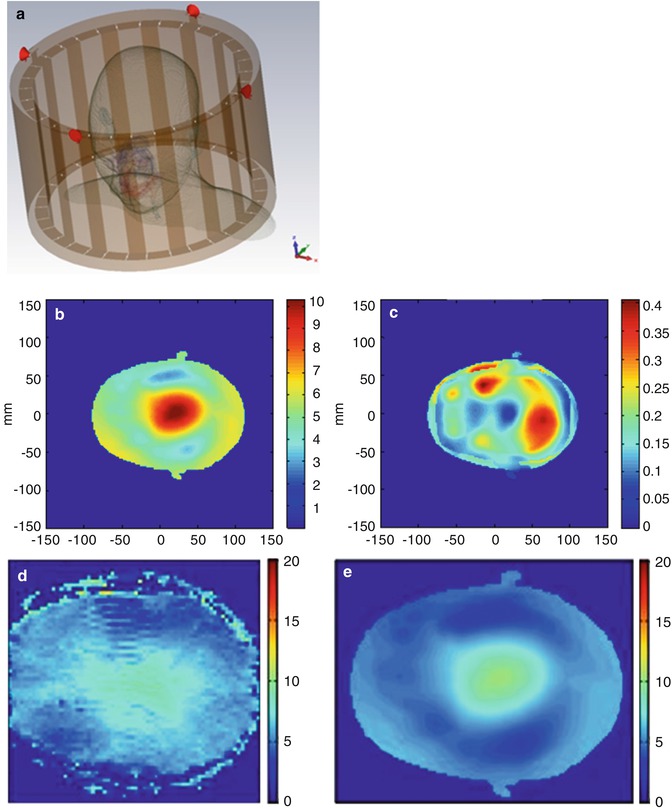
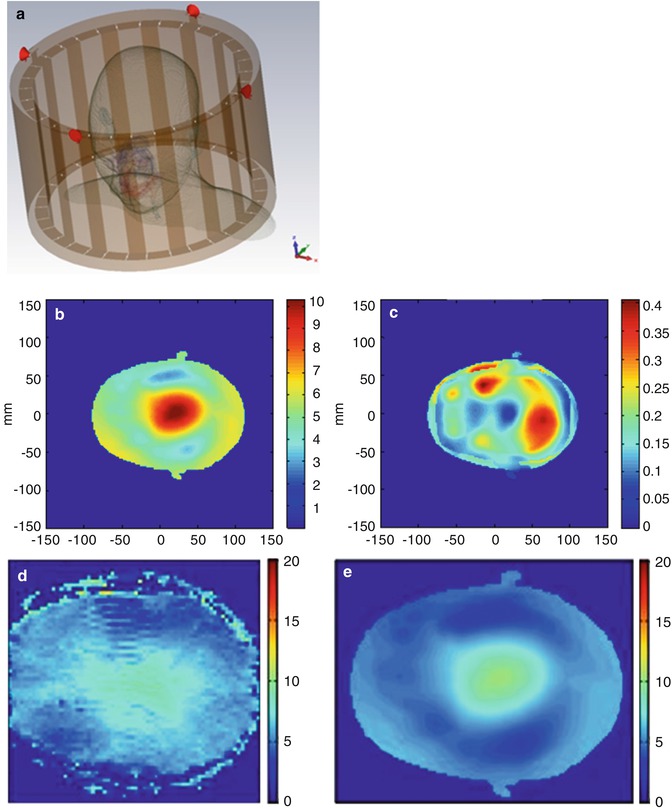
Fig. 23.2
The use of UHF MR in human needs a very careful safety assessment. In this context, calculating the specific absorption rate (SAR) becomes vitally important. SAR is a measure of the rate at which energy is absorbed by the tissue when exposed to radio frequency (RF) magnetic field per unit of mass. At ultra-high field, the distribution of the SAR within a patient becomes very uneven; this lack of uniformity is associated with the high-radio frequency (RF) output of the coil (298 MHz at 7 T). The calculation of RF fields and SAR is usually performed using full-wave electromagnetic simulators. In our case, we used the CST MW suite to simulate the volume transmitter in quadrature loading it with anatomical models of the human body (Hugo, Virtual Family, Virtual Classroom). In all the models, the voxel discretization has a resolution of 1 mm3, whereas the dielectric properties of tissue are taken from literature. Figure (a) shows Ella’s head (female Virtual Family model) within the volume transmitter in quadrature. Figures (b) and (c) show the map of B1+ (magnitude, in [μT]) and the SAR [W/kg] on the axial section passing through the eyes. Lastly, simply to validate the simulator, Figures (d) and (e) show the map of B1+ measured on a volunteer with characteristics similar to Ella [μT] and the appropriately scaled map of Ella [μT], respectively [11]
In recent years, huge technological progress has been made in attempting to resolve the problem of inhomogeneities in the excitation and in the receiving of RF signals in terms of both hardware and software. Rewriting the pulse sequence for UHF to enable the use of less sensitive sequences and eliminate errors in the flip angles applied to prevent sequence “refocusing” has proved extremely difficult. As the use of multichannel coils for signal receiving revolutionised MRI by allowing increased SNR and reduction of acquisition times, the introduction of parallel transmission opened new scenarios for UHF, creating a fundamental turning point in the use of UHF. Parallel transmission uses and controls a multichannel array system for the signal transmission, adjusting independently phase and amplitude of signals sent to each channel, with the aim of producing uniform excitation inside the sample. This led to what is now known as “RF shimming” (radio frequency calibration). Recently, parallel transmission systems allow to manage the different channels of signal transmission not only by adjusting the phases and amplitudes of signals but also by sending completely independent signals to single channels, increasing the spatial selectivity of the RF pulses. Thanks to this technological advance, it is possible to compensate specific B1 inhomogeneities induced by specific geometry and dielectric properties of each single human body exanimated. Corrections of B1 are therefore “customised” and allow the quality of imaging to be improved while at the same time minimising the depositing of energy for each patient with his or her specific characteristics (gender, age, dimensions such as weight and height, muscle mass and the presence of physiopathological signs).
As described above, some physical key UHF MR phenomena are not favourable for the application of 7 T in a clinical context such as inhomogeneities in B1 and the corresponding distribution of SAR. Moreover, the shortening of the RF wavelength used at UHF can interfere more strongly with metal objects increasing the heating effect. For this reason, the presence of implanted metal objects remains the main cause of exclusion from UHF MR exams.
With knowledge of above-mentioned limits and the significant results expected from awareness of the physical phenomena of UHF MR, the encouraging results of experimental studies carried out on patients suffering from diseases of the central nervous system have recently started to arrive.
23.2 Applications of UHF MR
From its first introduction into clinical practice in the 1980s, MRI has become a key tool for studying the anatomy and function of the central nervous system (CNS). At present, 7 T scanners around the world are used only for experimental purposes, and most of these experiments involve improving both hardware and software of the systems, in order to overcome the technological limitations intrinsic in the ultra-high field.
Studies about the applications to diseases of the CNS are still limited and are aimed to assess the diagnostic-clinical benefits of UHF with respect to clinical scanner, as well as to investigate safety aspects of application of UHF in humans (Fig. 23.3).
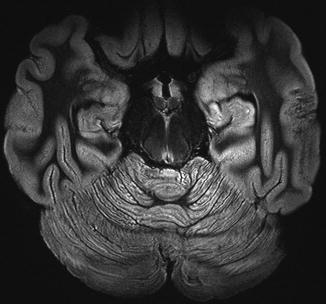
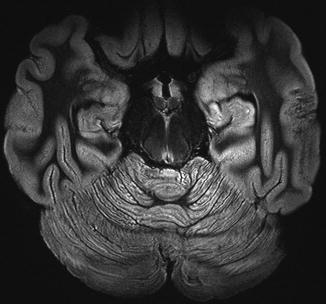
Fig. 23.3
Ex vivo 7 T cerebellum. Ex vivo imaging of the cerebellum at 7 T: a gross specimen from a human cadaver was fixed in a 10 % aqueous solution of formaldehyde, placed in a perfluoropolyether suspension and imaged with an IR sequence. The image resolution was about 100 microns and allows the appreciation of extremely fine details of the human anatomy such the cerebellar folia
The physical phenomena of UHF MR regulate the diagnostic possibilities obtainable with these scanners in a counteracting manner and provide benefits in some cases and disadvantages in others. The limited diffusion of UHF, the high costs involved and the presence of artefacts in the images limit its use in experimental studies, but at the same time push the development of new signal treatment methods and technological development. The possibility of obtaining new contrasts acts as a stimulus for the development of a new imaging semeiotics of the CNS in both healthy and unhealthy subjects. This is a true challenge for future clinical applications.
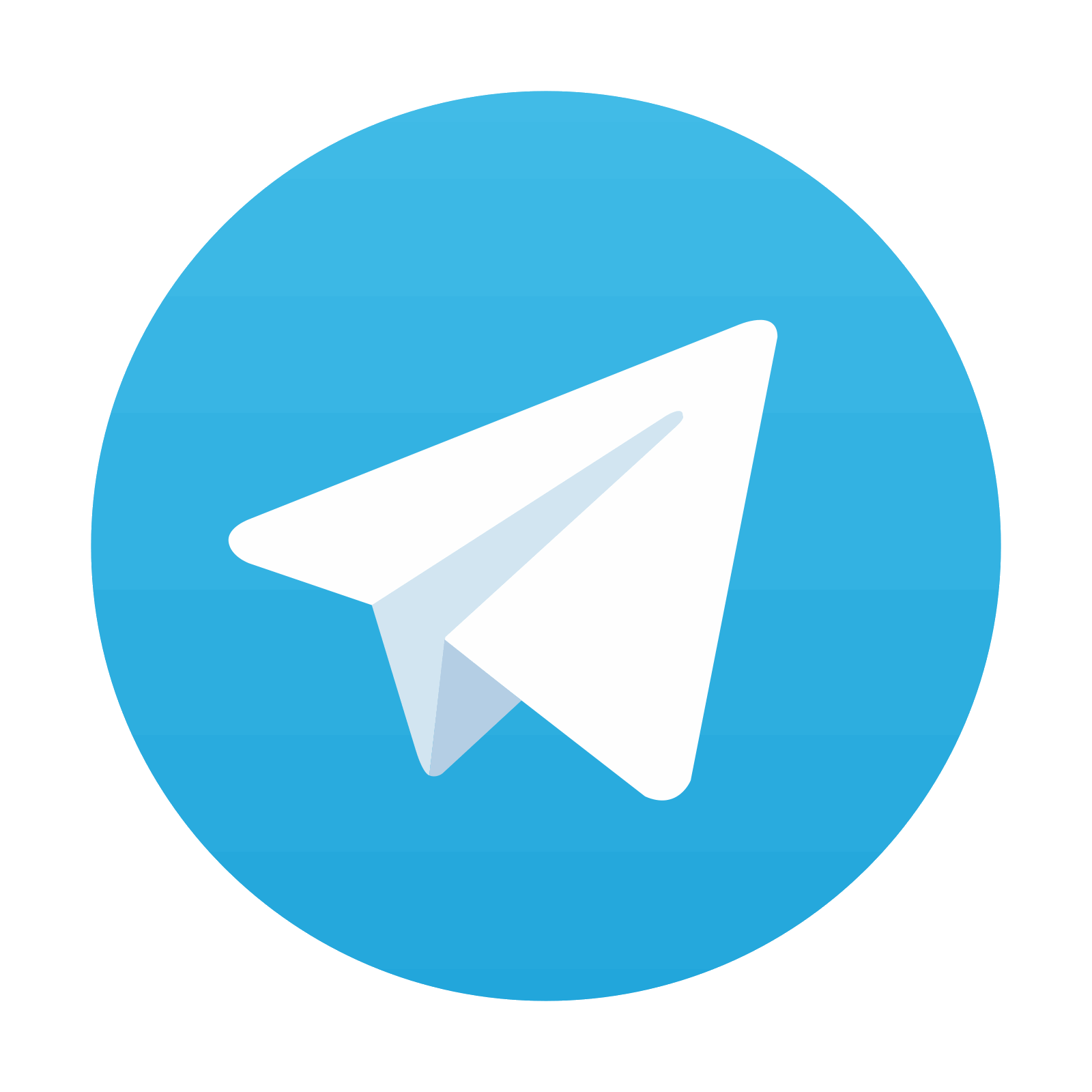
Stay updated, free articles. Join our Telegram channel
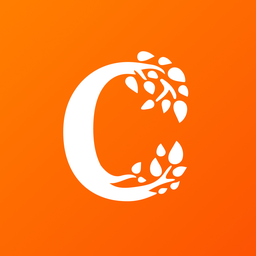
Full access? Get Clinical Tree
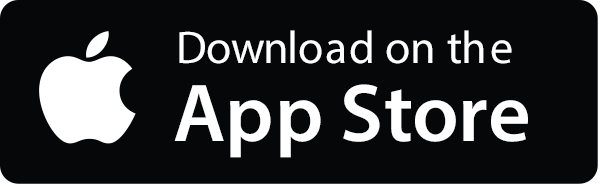
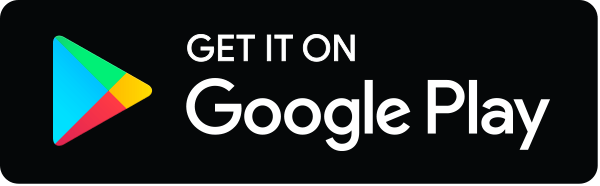