Fig. 21.1
An example of 3D reconstruction. Activation maps are superimposed on the high-quality volumetric acquisition, giving a better opportunity to localize eloquent areas. MPR projections are also shown
21.7 Software
The most commonly used packages are SPM and Brain Voyager; both import DICOM data, perform 2D and 3D statistical analysis and process single- or multi-subject data. Brain Voyager also has a real-time utility, Turbo Brain Voyager. There is no need to describe this well-known software in detail, suffice it to say that, at the beginning, fMRI was used only for research purposes and the software was targeted at this goal. With the increasing diffusion of this technique and its clinical application, manufacturers of magnetic equipment entered the market and began the production of new software. An example is the newly introduced GE software, BrainWave, which cannot process multi-subject data but is very convenient and has many advantages, so that we chose it as the software for fMRI analysis in the routine clinic. The package consists of two parts: real time and post-acquisition. Real time allows visualization of statistical t-maps during the acquisition; as the acquisition proceeds, statistical activation maps are processed and superimposed in real time on the fMRI series just acquired. This way, it is possible to monitor the situation and the activating effects from the very beginning of the acquisition. The post-acquisition package performs an in-depth analysis (similarly to Brain Voyager) but in a semiautomatic way; it may run in the background, during successive acquisitions or exams, and produces, in the final steps, two outputs: 3D-coloured activation maps and the so-called BIP maps, in which the activated areas, bounded by a white contour, are superimposed on the 3D structural isovolumetric sagittal acquisition. BIP maps are a DICOM output; they can be represented, in turn, in the three planes of the space (MPR) and, sent to the neuronavigator, are inestimable value to the neurosurgeon (Fig. 21.2).
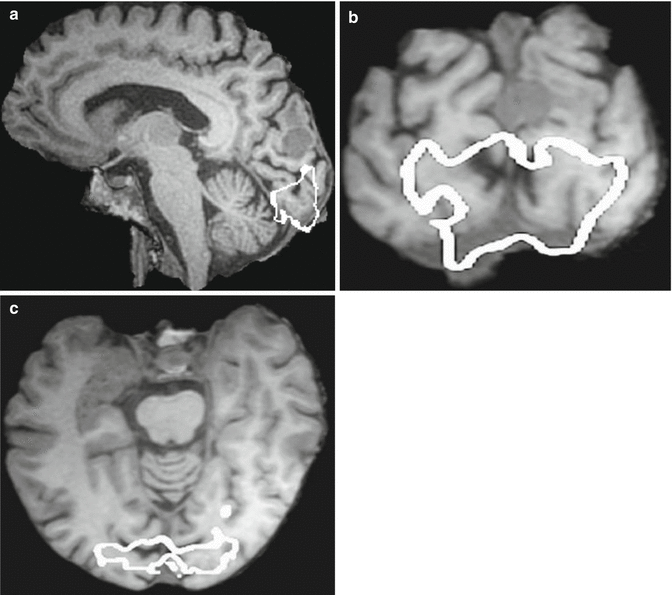
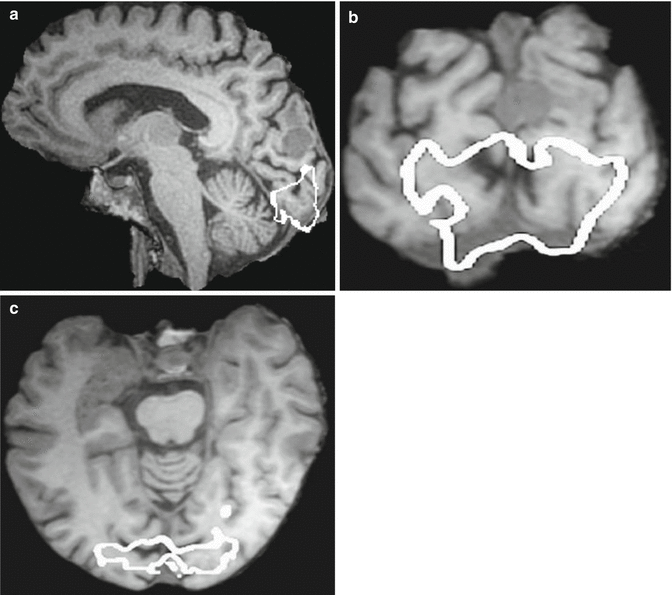
Fig. 21.2
An example of BIP maps. The activated areas in the occipital lobe are bordered by a white line, leaving the inner area transparent, to better define the anatomical structures involved (Modified from Leonardi et al. [33], with permission)
21.8 Paradigms
21.8.1 Motor Paradigms
Motor and sensory paradigms were the the first to be implemented [46], both because of the ease with which they can be performed in the scanner with no additional equipment and their easy validation by preoperative and intraoperative cortical monitoring. The good correlation between cortical monitoring and fMRI results made fMRI motor tasks the method of choice for the presurgical evaluation of patients.
There are many motor tasks described in the literature. Owing to the cortical distortion of the sensorimotor homunculus, the wider areas of fingers, hands, tongue and lips are the most frequently explored to produce the highest BOLD signal. Furthermore, hands/fingers and lips/tongue are very important anatomical effectors, involved, respectively, in day-to-day manual activities and speaking. As a consequence, hand and lip movements are the most often used tasks to explore the activity of the primary motor cortex. When the lesion is located near the convexity of the brain, movements of the foot are also used.
As regards the hand movements, there is a wide spectrum of paradigms ranging from the simple opening and closing of the hand or squeezing a sponge to the more complex sequential tapping of fingers in predetermined fixed order or repetitive opposition of the thumb and each of the remaining fingers. Simple and complex hand motor tasks bring about different cortical activation patterns.
Simple hand movements activate only the contralateral primary motor cortex, the superior part of the precentral gyrus, in an area called the “precentral knob”, otherwise named inverted Ω, which may also be divided by a sulcus in the middle and in that case is called horizontal ε [60, 61]. Complex hand movements activate not only the contralateral primary motor cortex, but also the ipsilateral motor cortex, the supplementary motor area, the premotor and the somatosensory cortex bilaterally [55]. There are different fundamental reasons for the primary sensory cortex activation. From the anatomical point of view, cytoarchitectonics show that pyramidal cells can be found either in the pre- or postcentral gyrus and motor fibres in the pyramidal tract originate not only from primary motor areas (MsI), but also from primary sensitive areas (SmI). According to classical neurophysiology, it is possible to elicit motor responses by electrically stimulating the precentral gyrus, as well as the postcentral gyrus, with a partial overlapping of the homunculi of cortical motor and sensory representation. Finally, both sensory proprioceptive and exteroceptive afferents can be activated by positional changes during the performance of motor tasks.
When the motor task is complex, there is also an asymmetry in lateralization, as regards the dominant hemisphere. In right-handed subjects, finger movements of the right hand substantially activate the dominant (left) hemisphere with almost no activation in the nondominant (right) hemisphere [29]. In left-handed subjects, the activation pattern may show a high degree of variability, but often both left- and right-hand movements produce activations comparable between dominant and nondominant hemispheres [50]. Obviously, all these differences must be taken into account in analysing an activation pattern.
Figure 21.3 shows an example of eloquent areas evoked by the repetitive sequential opposition of the thumb and each of the remaining fingers of the right hand.
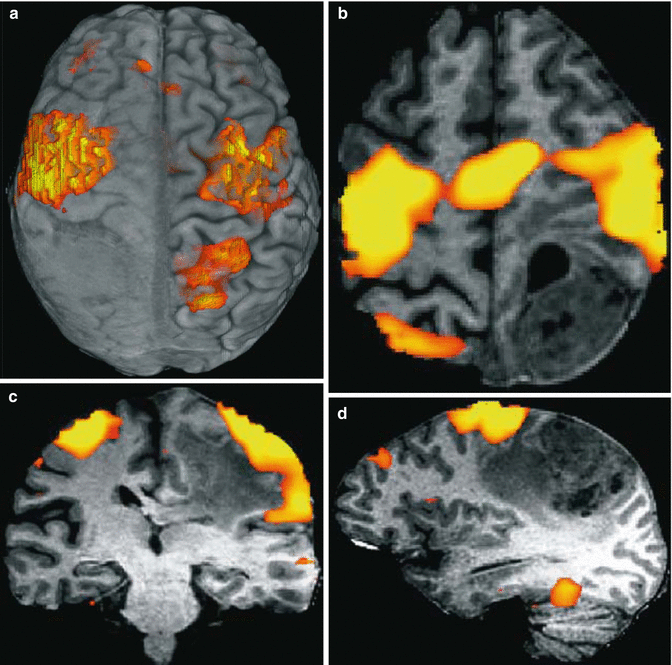
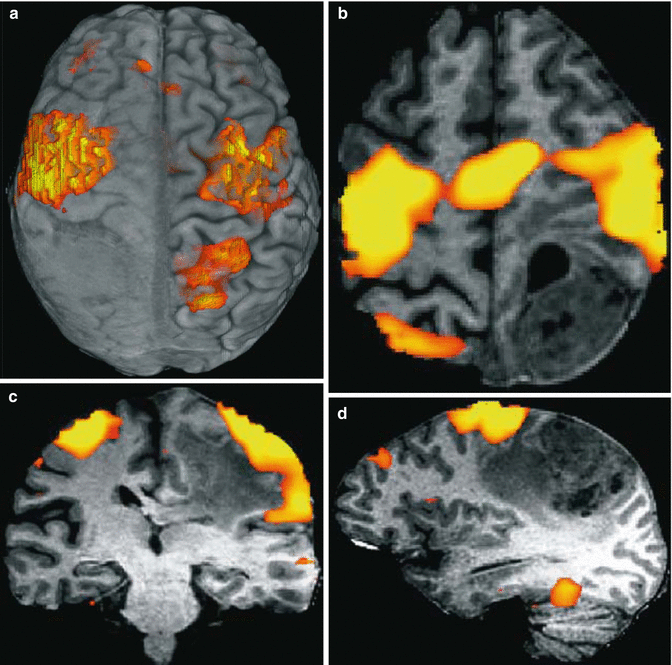
Fig. 21.3
An example of motion paradigm (repetitive sequential opposition of the thumb and each of the remaining fingers of the right hand). Note that activated areas are adjacent to the lesion (a meningioma). The patient underwent surgery with no sequelae
21.8.2 Sensory Paradigms
Sensory paradigms are used less often than motor paradigms but have the advantage of being a passive task, which may also be performed on uncooperative patients (anaesthetized, unconscious, neurologically impaired, disabled, aged, babies, etc.). In this last case, they could be the only way to identify the sensorimotor cortex for surgical planning.
The most common way to perform a sensory paradigm is by tactile stimulation of the skin of the hand or, less frequently, of the foot or face. Simple plastic toothbrushes, blunt nails, air puffs and even the examiner’s fingertips may accomplish this kind of stimulation.
Results show eloquent areas in both the postcentral and the precentral gyri [42]. The situation is similar to that which we have seen in motor paradigms: there is an anatomical cytoarchitectonic reason (i.e. the presence of granular cells not only in SmI but also in MsI) together with numerous connections through corticocortical or thalamocortical relays [59]; moreover, a direct cortical stimulation of the motor cortex in humans evokes sensory experiences [6]. The concept of a narrow, discrete, pre-Rolandic motor cortex separated from post-Rolandic sensory strip, although pervasive, has been challenged by evidence of a broad overlapping sensorimotor cortex [54].
21.8.3 Visual Paradigms
Before the introduction of fMRI, the most common way to obtain functional information about important anatomical visual areas, such as the fibre system of the optic radiations, the lateral geniculate nucleus and the striate/extrastriate cortices, was by perimetric examination of the visual field. This technique, however, provides only a subjective determination, at each point, of the functional variation in time and lacks direct anatomical information. Conventional neuroradiological imaging, on the other hand, simply outlines lesion location and its gross extent, but it is often difficult to establish if the presence of oedema or structural alterations of local anatomy imply neuronal death. A better understanding of the function-structure correlation of striate organization has been provided by functional PET imaging studies, but the associated radiation exposure and the limited availability of PET units have restricted its application for routine evaluation of patients with visual field defects to those described in a few clinical reports [30].
The enhanced spatial and temporal resolution of non-invasive fMRI, together with its safety and availability, has provided new and valuable information in understanding the organization and functional properties of visual areas in the human cortex. For instance, fMRI has been a precious method in confirming cortical retinotopy and quantitatively defining cortical extension of the central foveal vision [30, 48], results that, otherwise, could only have been obtained in an invasive manner. fMRI imaging can detect objective visual field deficits, caused by lesions not only producing destruction of the primary visual cortex, but also interrupting visual pathways and creating a lack of sensory input, without destroying neurons in the occipital cortex [30].
Visual stimulation may be delivered in plenty of modes: frequently used stimuli are flashing of white or coloured lights at certain frequencies or alternating checkerboards, stripes or bands. Stimuli may be presented by a video screen or by goggles. This last device is often preferred, because it allows different kinds of stimulations (e.g. monocular, different mixes of hemifields, quadrants) and allowed us to explore cortical visual retinotopy. Moreover, it provides a better concentration for the patient, who cannot see anywhere but into the goggles. The resting condition is frequently characterized by a black screen with a fixation point in the centre. We implemented visual paradigms in our department by using goggles and alternating (500 ms period) black and red vertical bands (Fig. 21.4).
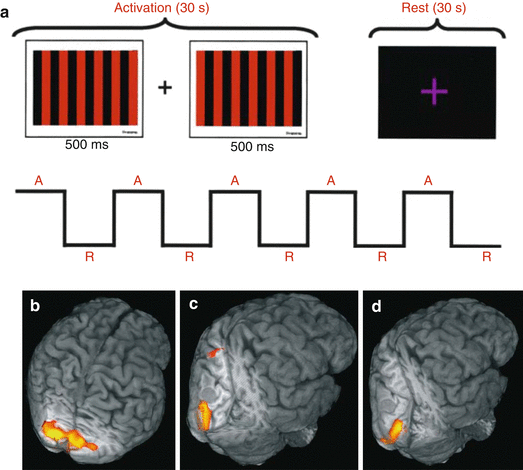
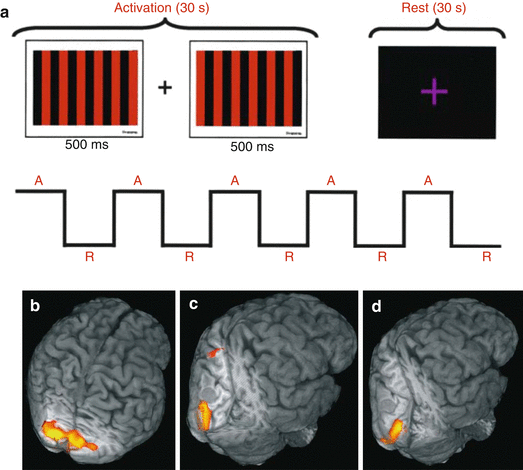
Fig. 21.4
An example of visual paradigm. (a) Stimulation (alternating black and red vertical bars with a period of 500 ms) and rest alternate every 30 s. Stimulation may involve full visual fields bilaterally (b) or superior/inferior right quadrants (c, d, respectively). Cortical retinotopy is respected. Note the small meningioma on the left hemisphere (Modified from Leonardi et al. [33], with permission)
21.8.4 Language and Lateralization Paradigms
Since the classical works of Wernicke and Broca, the location and definition of brain language areas have been a goal and a challenge for researchers. Language areas have traditionally ascribed to two discrete regions: Wernicke’s area, which is responsible for the receptive aspects of language (comprehension), and Broca’s area, which controls the expressive aspects of language (production). The former is located in the left posterior temporal lobe and the latter in the left inferior frontal lobe, anterior to the central fissure; both are interconnected by the arcuate fasciculus that allows information exchange between the two.
Language areas are usually located in only one hemisphere, more frequently the left, but lateralization may not remain constant: translocation of single Wernicke’s [42] or single Broca’s [24] areas to the contralateral hemisphere has been demonstrated in right-handed patients, when, for instance, a slowly growing tumour allows brain plasticity mechanisms to come into play.
Owing to the complexity of language-related functions, the exact location of these functional areas is somewhat variable and cannot be predicted on the basis of anatomy alone. Of course these areas are of great importance to the private and social life of the patient, and sparing them is essential when a surgical approach is required.
Before the arrival of PET and fMRI and for approximately half of the previous century, the Wada test represented the “gold standard” and the task routinely used to assess the language-dominant hemisphere. Briefly, the Wada test requires the catheterization of both the internal carotid arteries and the successive injection, for each side, of amobarbital (125 mg), followed by hemispheric anaesthetization, to exclude one hemisphere at a time; the final step is the study of the awake hemisphere to establish persistence or disruption of language functions. Of note, the Wada test is possible only when there are no vascular abnormalities allowing arterial crossflow between the two hemispheres.
The advent of fMRI has changed the approach to language exploration. As one can easily infer, the Wada test has many disadvantages with respect to fMRI: invasiveness, higher risk, higher cost and very short amounts of time (5–10 min) available to explore the awake hemisphere. fMRI, in addition to being non-invasive and cheaper, is not affected by underlying supply patterns; can be easily repeated, if necessary, without additional risk; and affords the examiner sufficient time to test a range of cortical functions. Moreover, owing to the fact that the entire brain is examined simultaneously, results are not confounded by difficulties in reproducing test conditions separately for each hemisphere, as in the Wada test. As a consequence, several studies have compared fMRI to the Wada test and have shown a significant correlation between results from both modalities in most cases. fMRI studies typically identify more language regions than direct electrocortical stimulation mapping and the Wada test, suggesting that fMRI not only identifies areas that are critical for language processing but also areas that participate in a less critical manner in networks that sustain language function. The result is a wider availability of information.
Plenty of paradigms have been used in the literature (cf. [7] for an exhaustive review) to define lateralization and locate language areas. On the whole, language paradigms may be classified into two main categories: the ones exploring receptive language and the ones exploring expressive language.
Receptive Language Paradigms
This kind of task explores the comprehension aspect of language and elicits eloquent areas corresponding to Wernicke’s areas proper (the posterior part of the superior temporal gyrus, posterior BA22) and the surroundings (the anterior-superior and middle temporal gyri, anterior BA22, the mid-superior temporal sulcus, midportion of BA21 and BA22), with the adjacent angular (BA39) and supramarginal (BA40) gyri, in the dominant hemisphere.
Receptive language paradigms are mainly divided into reading comprehension (visual input) and auditory comprehension (auditory input). The most common reading comprehension task consists of reading stories, in which each paragraph is presented at a fixed interval of time, according to the reader’s speed. The most common auditory comprehension task consists of listening to stories. The latter is a passive task, which, like sensory paradigms, can also be performed on uncooperative patients.
When visual input is used, one has to consider brain activation induced by visual input, in addition. This activation does not concern the primary visual cortex only but also the ventral extrastriate inferior temporal/occipital regions, involved in transferring the modulated inputs to Wernicke’s area. Otherwise, when auditory input is used, this will produce an auditory response in the adjacent auditory cortices of the superior temporal gyri. To isolate Wernicke’s area and delete visual/auditory responses, other visual/auditory stimuli (e.g. uppercase/lowercase letters or backward text, respectively) may be used in the contrasting control task. However, this is not the case for presurgical purposes, because auditory/visual areas also have to be spared from the surgery.
Figure 21.5 shows an example of receptive language paradigm (listening to a reading).
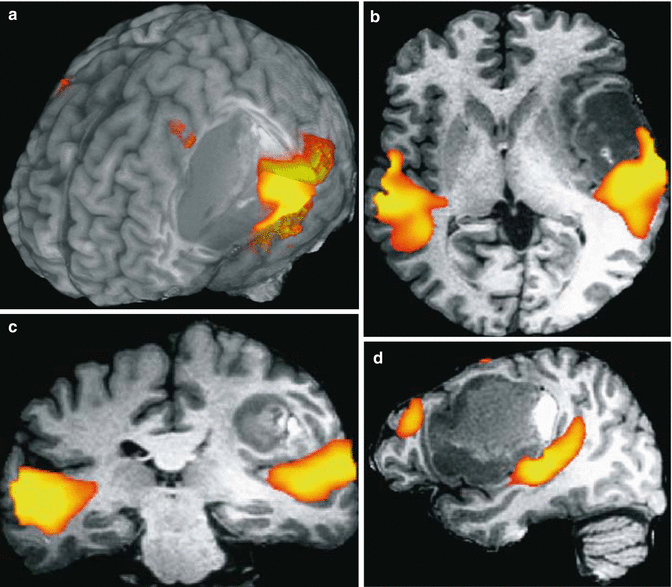
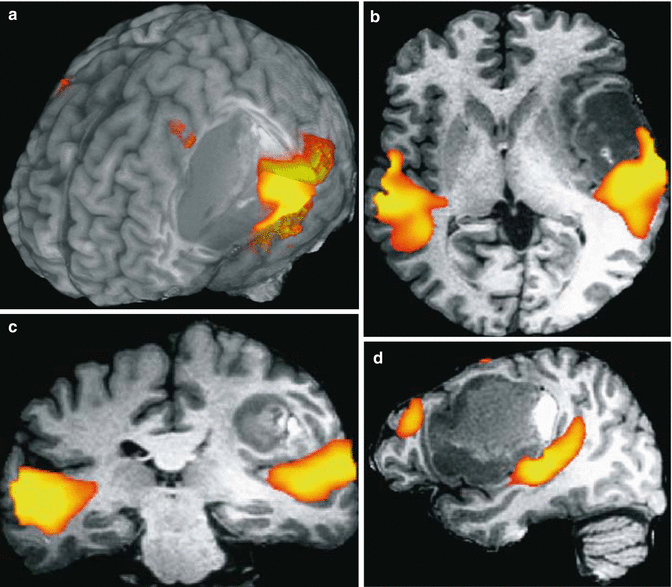
Fig. 21.5
An example of receptive language paradigm (listening to a reading). Note that the activated areas are very adjacent to the lesion (glioma) and intermingled with it. The patient underwent surgery and had postsurgical deficits (aphasia)
Expressive Language Paradigms
This kind of task explores the production aspect of language and elicits eloquent areas corresponding to Broca’s area proper (the pars opercularis, BA44, and the posterior portion of the pars triangularis, posterior BA45, of the inferior frontal gyrus), plus the precentral gyrus of the insula, in the dominant hemisphere.
Most of the numerous existing paradigms can be classified into two main categories: verbal fluency and semantic decision. The main difference between the two is that semantic decision tasks do not only explore language production only but also some working memory functions; the result is the activation of other areas in addition to expressive language ones (see below).
Verbal fluency tasks rely on the ability to produce words in different ways. Worth noting is that the patient is requested to generate words covertly (inner speech), to avoid possible artefacts caused by movements of the lips, tongue and head in active word generation. When the task is of the phonological kind, the patient is visually or verbally cued with a letter of the alphabet at the beginning of each task period and asked to think of as many words as possible that begin with that letter. Of course, the letters change in each task period. When the task is of the semantic kind, the patient is visually or verbally cued with a certain category (e.g. animals, flowers) at the beginning of each task period and asked to think of as many words as possible that belong to that category. During the rest condition, the patient may repeat a single word (e.g. “bla”, “bla”, “bla”) or may think of nothing. Receptive language areas are activated by both paradigms, but the phonological task gives a more clear-cut activation than the semantic one [44].
A verbal fluency task, which is very well used and reliable in disclosing lateralization, is verb generation from words. A sequence of words is aurally or visually presented to the patient, who has to think of a verb corresponding to each word (e.g. piano → to play), whereas the rest condition consists of silence. In addition to Broca’s area, other eloquent areas may be activated in the dominant hemisphere: the pars orbitalis of the inferior frontal gyrus, BA47; the middle frontal gyrus, BA46 and 9; and the prefrontal and posterior temporal cortices.
Semantic Decision Tasks
In this kind of task, a couple of words are sequentially presented to the patient, who has to decide if the couple appertain to a certain semantic category stated previously (e.g. synonyms vs antonyms, abstract vs concrete, living vs nonliving). Rest conditions differ according to whether the task is given aurally or visually. In the first case, couples of tones are presented, and the patient has to decide if they are identical or not. In the second case, couples of letter are shown, and the patient has to decide if they are written in uppercase or in lowercase. As already mentioned, these tasks activate other areas, besides Broca’s area, and these are the middle and the inferior temporal gyri, fusiform and parahippocampal gyri, the cingulate cortex (BA32) and the superior frontal region (BA8) in the dominant hemisphere.
21.9 Presurgical Applications of fMRI
21.9.1 fMRI and Brain Tumours
Certainly, fMRI takes pride of place in the presurgical evaluation of brain tumours. As stated in the introduction, fMRI is of inestimable value in locating eloquent areas when normal relationships between anatomy and function are lost (e.g. when a mass effect distorts the anatomical landmarks or when functional areas may be relocated to other areas in the brain). However, in some situations, fMRI activation in and around tumours must be interpreted with caution.
Schreiber et al. [47] found that fMRI activation is reduced near glial tumours, but is usually not affected by non-glial tumours. They suggested that this phenomenon might be explained by the fact that glial tumours grow in a more infiltrative manner and thus alter the cellular architecture, whereas non-glial tumours show more delineation from normal tissue, leaving the cellular architecture intact.
Primary brain tumours, especially low-grade tumours, have been shown to have functional tissue preserved within the lesion itself. Negative findings may represent language cortex working but having an activity level below the sensitivity of the technique and thus not being detected. Moreover, it is known, from both angiographic and MR studies, that tumour vasculature in malignant gliomas loses the ability to autoregulate. If the brain’s ability to autoregulate the flow of blood is lost in brain tissue, which is still functioning, then this area may not respond to increased neural activity by a corresponding increase in blood flow [25]. Another reason adduced by the same authors is the mass effect. Venous structures are normally under low pressure and are easily compressible. The increased tumour mass effect compresses the venules and larger veins, thereby speeding the egress of deoxyhaemoglobin-laden blood from the area of activation. This leads to a decrease in the relative concentration of deoxyhaemoglobin in the area of activation, which in turn results in an effective decrease in the difference in concentration of deoxyhaemoglobin between the resting and active states. This would lead to a decreased ability of fMRI to detect changes between the resting and active states [25].
However, intraoperative cortical mapping confirmed most of the fMRI findings and showed that the possible limitations of technique concerned only some selected cases and did not impede the successful identification of the eloquent cortex in the vast majority of patients of this kind.
21.9.2 fMRI and Epilepsy
Another important field of fMRI application is the presurgical evaluation of patients with refractory epilepsy. It is well known that about 90 % of well-selected patients become seizure-free after surgical treatment [17]. As far as the surgical outcome is concerned, fMRI becomes an important tool for selecting and better characterizing these patients.
fMRI in fact plays a significant role not only in defining the lateralization of language functions but also in localizing the specific language areas. As to this last point, it has been shown that patients with epilepsy tend to have more “bilateral” activation: they reveal a significantly higher recruitment of contralateral homologous language areas, compared to the normal controls. Moreover, the earlier the onset of dominant temporal lobe seizure foci, the more widespread and atypical the distribution of language areas; expressive and receptive language skills can also dissociate in people with brain lesions occurring early in life [2]. In this context, fMRI becomes an invaluable help in mapping clinically relevant language functions in the epilepsy surgery population.
Another advantage of fMRI use is the possibility of defining the seizure spatially and temporally. Krings et al. [31] performed fMRI on a patient, who happened to experience a simple partial seizure; the seizure was associated with changes in MR signal in different regions, showing the spatiotemporal course of spreading. fMRI data correlated with EEG-determined seizure foci. Hence, it is possible to use fMRI not only to detect the cortical location of activations associated with the seizure but also to define the epileptogenic focus in the originally activated area. The recent development of EEG-triggered fMRI allows interpretable electroencephalographic data to be recorded during MRI scanning. In this way, it is possible to combine the spatial resolution of MRI with the temporal resolution of electrophysiology in the seizure localization. EEG-linked fMRI acquisition is a promising technique in the field of epilepsy.
21.9.3 fMRI and AVM
The presurgical evaluation of arteriovenous malformations (AVMs) is another interesting and controversial application of fMRI. They are the most common of cerebrovascular malformations and consist of a coiled mass of arteries and veins, without an intervening capillary network, lying in a bed formed by displacement rather than invasion of normal brain tissue. Functionally, they are direct arteriovenous communications causing a shunt of blood from the arterial to the venous side. The high flow volume shunted through an AVM fistula appears as “voids” within the structure in morphological MR images and may induce a decrease in cerebral perfusion pressure in the downhill artery [37].
The surgical importance of AVMs is related to their high probability of bleeding and giving epileptic crisis. In such situations, the localization of eloquent cortices near AVMs becomes important not only presurgically but also during possible embolization procedures, because, when there is doubt that an AVM is close to eloquent tissue, particular care must be exercised to avoid devascularizing these functional areas.
The debate about the use of fMRI in AVMs revolves around the peculiar haemodynamics of this pathology. AVMs produce high-velocity, low-resistance blood flow and induce feeding artery hypotension and draining vein hypertension, with a potential net reduction in cerebral perfusion pressure in neighbouring territories. Chronic hypotension does not necessarily result in loss of neuronal function in brain tissues surrounding AVMs, but haemodynamic perturbations may reduce or impede the BOLD signal in adjacent eloquent cortex, obscuring activation where neuronal function may be present.
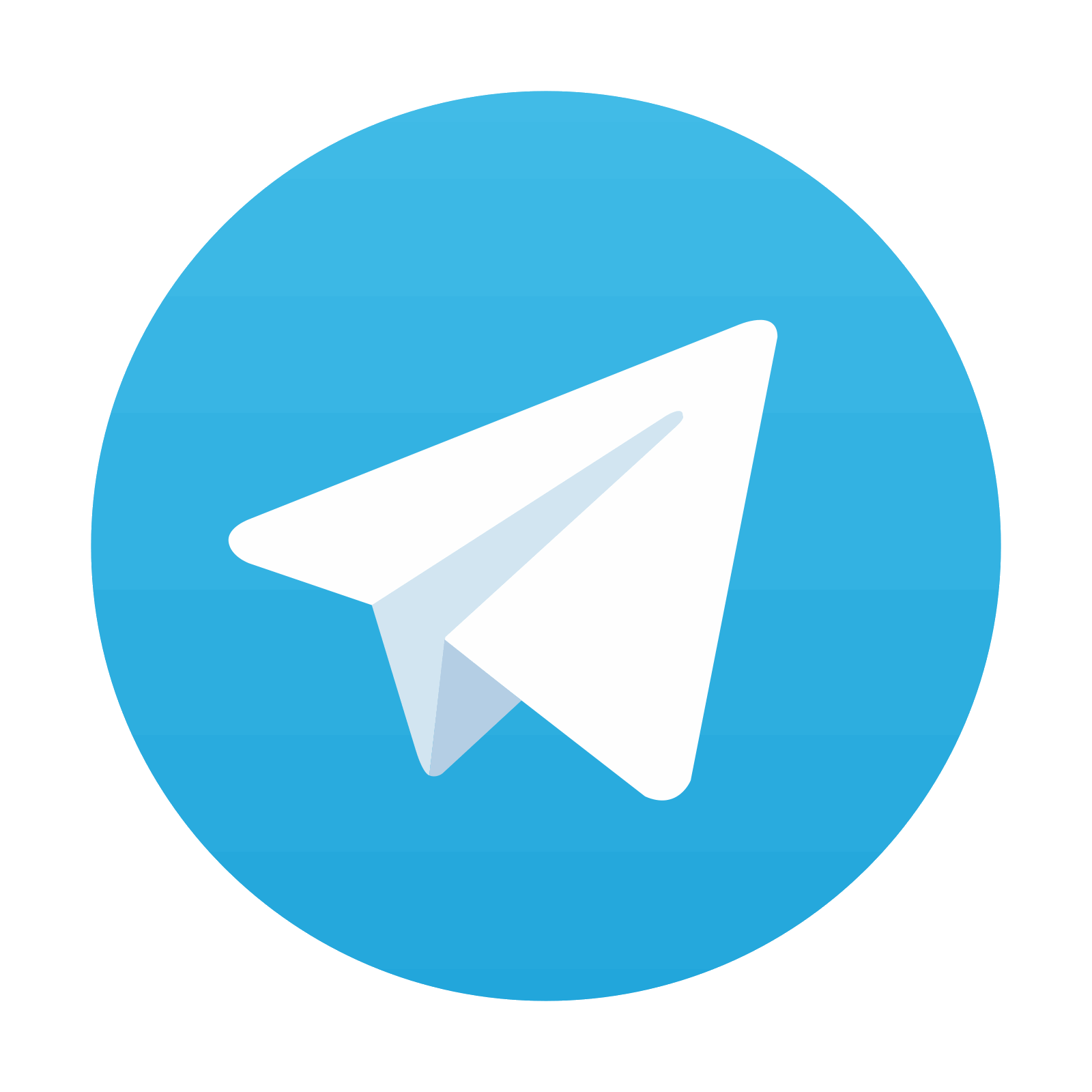
Stay updated, free articles. Join our Telegram channel
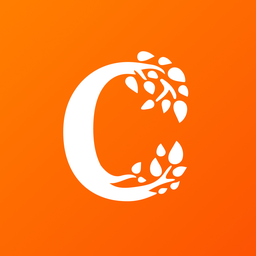
Full access? Get Clinical Tree
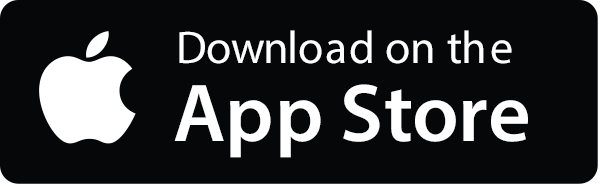
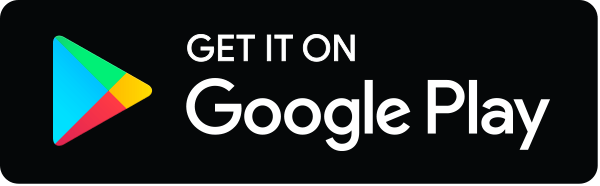