Fig. 10.1
Arterial spin labeling basic principle. The magnetization of water protons is inverted within a thick slab covering feeding arteries at the base of the head. After a time delay, inserted to allow labeled spins to reach the brain regions of interest, an image is acquired (M L, “label” image). After a few seconds, the image is acquired again without the labeling process (M C, “control” image). Non-labeled spins are represented as upward vectors and the labeled spins as downward vectors. In the label image the number of spins with inverted magnetization will vary according to local blood supply
Perfusion-weighted images can be generated by the difference between unlabeled and labeled images. In practice, the ASL difference signal (MC – ML) is a small fraction (about 1 %) of the source image signals, requiring collection of multiple image pairs and averaging to provide adequate signal-to-noise ratio [7]. An example of ASL perfusion-weighted image obtained on a young healthy subject acquiring 35 control-label pairs is shown in Fig. 10.2.
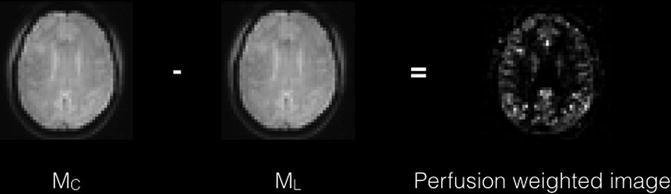
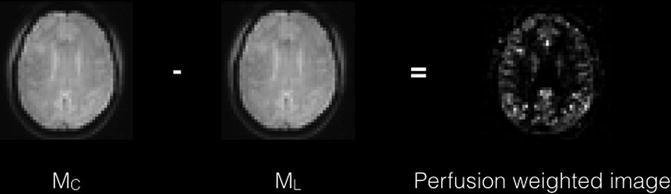
Fig. 10.2
The magnetization difference between control and label images is proportional to the regional blood flow, since the contribution of static spins cancels out
10.3 ASL Sequences and Labeling Schemes
Since the original introduction of the basic ASL technique [15, 16], numerous ASL sequences have been developed. The main classification is based on how labeling is achieved, with sequences categorized as either continuous ASL (CASL) or pulsed ASL (PASL), with their own pros and cons. An example of the two labeling methods is shown in Figs. 10.3 and 10.4.
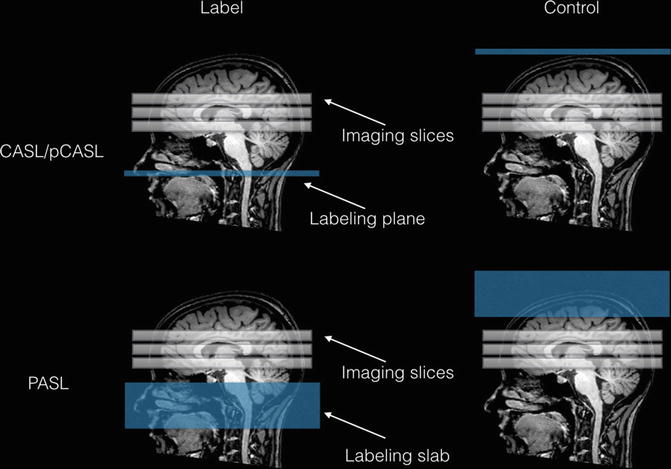
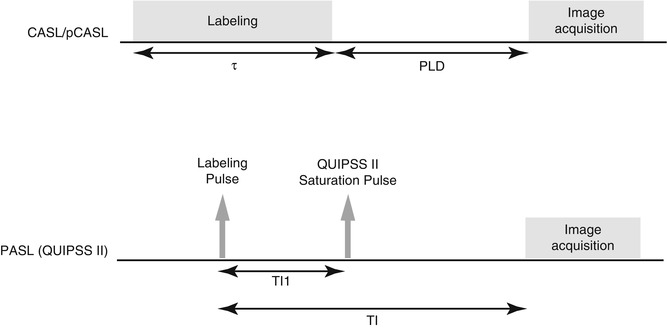
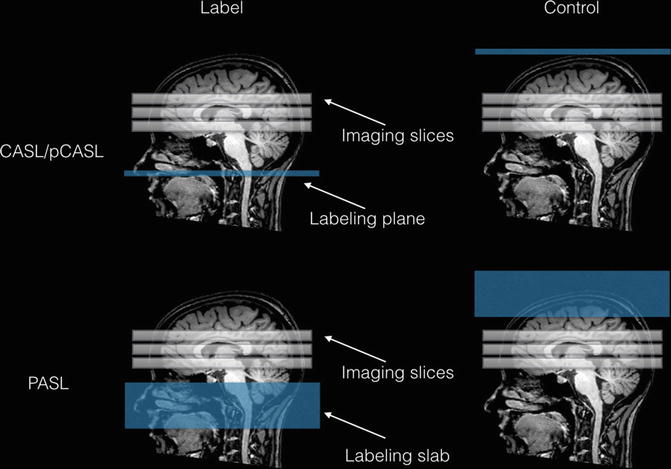
Fig. 10.3
Schematic representation of the continuous (CASL) and pulsed (PASL) labeling approaches. In CASL, the magnetization of flowing blood is inverted as it crosses the labeling plane, creating an arterial bolus of magnetically labeled blood with a duration equal to the duration of the RF pulse. To compensate off-resonance effects of the long labeling RF pulse, an analogous pulse is applied in the control image on the distal side of the imaging slices, so that no arterial blood is inverted. In PASL, labeling is performed using a single short (~ few ms) RF pulse that invert arterial blood magnetization in a thick slab. As for CASL, off-resonance effects are compensated in the control image applying an inversion slab above the imaging slices
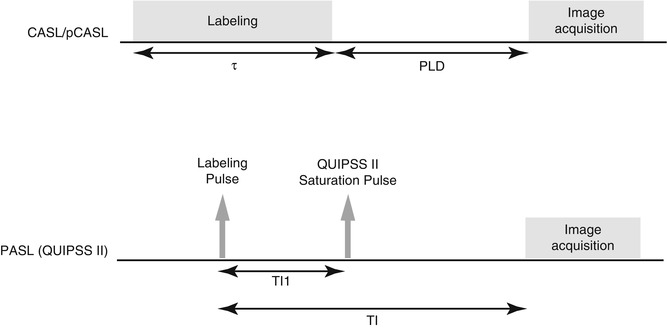
Fig. 10.4
Schematic diagram of the two ASL sequences, illustrating the labeling parameters described in the main text
Continuous ASL (CASL)
In CASL, the magnetization of arterial blood is continuously inverted in the feeding arteries using a combination of a continuous radiofrequency (RF) pulse and a gradient field that define an inversion plane, through a process called adiabatic fast passage [16, 30, 31]. The magnetization of flowing blood is inverted as it crosses this plane, creating an arterial bolus of magnetically labeled blood with a duration equal to the duration of the RF pulse. After the RF pulse is turned off, a post-labeling delay (PLD) is inserted, and the label image is acquired. In the CASL approach, the labeling duration is relatively long (~2 seconds), allowing the creation of a well-defined bolus and a good signal-to-noise ratio. However, a drawback of the long labeling RF pulse used in CASL is represented by its off-resonance influence on the acquired image due to magnetization transfer effects, usually causing signal loss [32]. If these spurious effects are not balanced in the control images, the difference (M C – M L) would reflect not only the blood flow but also the signal loss due to magnetization transfer effects that are present in ML but not MC. Different strategies have been developed to balance the magnetization transfer effects on both MC and ML images [15, 33]. The basic idea is to apply a RF pulse for the control image as well, designed with the same duration as the labeling pulse but applied above the imaged slices so that no inversion is produced on arterial blood (Fig. 10.3). A factor that limited the diffusion of CASL is that the continuous RF pulses are very hardware-demanding and are not supported on most clinical MRI scanners.
Pulsed ASL (PASL)
In PASL, labeling is performed using a single short (~few ms) RF pulse that inverts arterial blood magnetization in a thick slab. PASL has been implemented with many labeling schemes, differing on how the labeling slab is positioned in control and label images [34]. Here, the early implementation known as EPISTAR (echo-planar imaging and signal targeting with alternating radiofrequency) [35, 36] is described to illustrate the concept. With EPISTAR the labeling band is positioned proximal to the imaging slices, with a small (~1 cm) gap (Fig. 10.3). After a delay to allow the labeled blood to flow into the brain regions, the label images are acquired. As with CASL, the compensation of unwanted effects of the labeling process on the ASL difference image is critical and is accomplished with a proper acquisition of the control image. As shown in Fig. 10.3, the control image is acquired with an inversion pulse applied on a slab placed on the other (distal) side of the imaging slices. Ideally, this control pulse does not label any arterial blood that will flow into the imaging slices but produces off-resonance effects on the static spins similar to those produced by the labeling pulse. Note that with this scheme, venous blood entering from above could actually be labeled by the control pulse and so will appear as focal dark spots, corresponding to veins, in the ASL subtraction image. Unlike CASL, in this basic EPISTAR scheme, there is no control on the duration of the labeled arterial blood bolus. Indeed, in this implementation of pulsed ASL, the bolus duration is determined by the time required for all of the labeled blood to leave the labeling slab, thus depending on the subject physiological state that could influence blood velocity in the feeding arteries of the brain. While this poorly defined arterial bolus has negligible effects on qualitative perfusion-weighted images, it is a critical factor for quantification of CBF in physiological units. In order to control the duration of the arterial bolus of labeled spins, a modified PASL technique known as QUIPSS II is applied [37]. With QUIPSS II, a 90° saturation pulse is applied to the labeling band after the inversion pulse and before the image acquisition. In this approach, the inversion pulse is applied at t = 0, the saturation pulse is applied at TI1, and the image is acquired at TI. The saturation pulse is applied to the control image as well to balance off-resonance effects in the ASL signal difference. If TI1 is less than the time required for all of the labeled spins to leave the labeling band, the effect of the saturation pulse is to cut off the end of the arterial bolus, thus producing a well-defined bolus with a duration of TI1.
Pseudo-Continuous ASL (pCASL)
This approach uses a train of short RF pulses rather than continuous RF to invert the arterial blood spins [38]. Pseudo-continuous ASL has been developed to merge the CASL superior signal-to-noise ratio and the PASL high labeling efficiency without the need of particular demanding hardware [39, 40]. Indeed, the pCASL is emerging as a powerful tool for clinical studies, allowing good reproducibility and reliability with acquisition times of the order of a few minutes and a widespread availability across vendors [21, 41, 42].
10.4 Technical Advancements and Readout Strategies
Developments of ASL techniques are growing rapidly, expanding on the original concept to improve signal-to-noise ratio (SNR) and minimize confounding factors. One important addition is represented by the introduction of background suppression pulses that aim at suppressing the static tissue signal in order to improve the SNR of the ASL difference signal [43, 44]. As mentioned before, the signal of interest in ASL is the small difference of two large signals (Mc-ML). Systematic errors on these signals can be as large as the difference signal, thus biasing the CBF estimate. Since both MC and ML signals receive the main contribution from static tissue spins, suppression of this background signal can improve the quality of the difference signal. This suppression is achieved with carefully timed spatially selective inversion pulses that are applied to the imaging region prior to the excitation pulse for image acquisition, nulling the static tissue signal over a reasonably wide range of T1 values.
Both three-dimensional (3D) and two-dimensional (2D) readout schemes are applied for ASL image acquisition. For 3D, segmented methods such as RARE or GRASE [45–47] are mostly used, providing optimal SNR and low sensitivity to field inhomogeneity. Since 3D readout approaches require only one excitation pulse per volume, background suppression can be made highly effective, timing the inversion pulses to null the static tissue signal at the excitation time.
For 2D, single-shot EPI and spiral methods are commonly used, with similar performance to one another. Background suppression is less effective for 2D imaging, since the timing of suppression pulses will only be optimal for one or a few slices. However, the residual signal after the partial background suppression can be used for image registration prior to MC-ML subtraction, reducing the effect of motion artifacts on the ASL difference signal. In general, a short echo time is recommended to reduce T2/T2* weighting, and parallel imaging can be used to achieve both shorter echo times and reduced image distortions in 2D EPI [41]. The application of vascular crushing gradients has also been proposed to reduce vascular artifacts. If the arterial transit time is larger than PLD/TI, bright vascular signals will be evident in the ASL difference image. Vascular crushing gradients work by dephasing the signal from labeled spins that are still present in larger arteries at the time of imaging, thus reducing this type of artifact. This option can be useful in certain applications when large intravascular ASL signals may hide more subtle perfusion-related signals of interest, such as in tumors. However, in other clinical applications, bright intravascular signals due to increased arterial transit time may be a useful indicator, as in the setting of collateral flow and in general for arteriovenous malformation investigations, discouraging the use of vascular crushing gradients in these cases [48–50].
10.5 Absolute CBF Quantification
One advantage of the ASL technique is its ability to quantify perfusion in physiological units. In most cases a single PLD/TI ASL sequence is used, with CBF quantification based on a simple model [37, 41, 51]. The basic assumptions of this model require that (i) the entire labeled bolus is delivered to the target tissue, (ii) there is no outflow of labeled blood water, and (iii) labeled spins relax with blood T1 prior image acquisition. With these assumptions, voxel-wise CBF maps in physiological units can be calculated using the following expressions for CASL/pCASL and PASL (QUIPSS II), respectively [37, 51]:
![$$ CBF=\frac{6000\lambda \left({M}_{\mathrm{C}}-{M}_{\mathrm{L}}\right){e}^{\frac{PLD}{T_{1 A}}}}{2\alpha\ {T}_{1 A}{S}_{PD}\left(1-{e}^{-\frac{\tau}{T_{1 A}}}\right)}\left[ ml/100\mathrm{g}/ \min \right] $$](https://radiologykey.com/wp-content/uploads/2017/09/A128043_2_En_10_Chapter_Equ1.gif)
![$$ CBF=\frac{6000\lambda \left({M}_{\mathrm{C}}-{M}_{\mathrm{L}}\right){e}^{\frac{ T I}{T_{1 A}}}}{2\alpha\ T{I}_1{S}_{PD}}\left[ ml/100\mathrm{g}/ \min \right] $$](https://radiologykey.com/wp-content/uploads/2017/09/A128043_2_En_10_Chapter_Equ2.gif)
![$$ CBF=\frac{6000\lambda \left({M}_{\mathrm{C}}-{M}_{\mathrm{L}}\right){e}^{\frac{PLD}{T_{1 A}}}}{2\alpha\ {T}_{1 A}{S}_{PD}\left(1-{e}^{-\frac{\tau}{T_{1 A}}}\right)}\left[ ml/100\mathrm{g}/ \min \right] $$](https://radiologykey.com/wp-content/uploads/2017/09/A128043_2_En_10_Chapter_Equ1.gif)
(10.1)
![$$ CBF=\frac{6000\lambda \left({M}_{\mathrm{C}}-{M}_{\mathrm{L}}\right){e}^{\frac{ T I}{T_{1 A}}}}{2\alpha\ T{I}_1{S}_{PD}}\left[ ml/100\mathrm{g}/ \min \right] $$](https://radiologykey.com/wp-content/uploads/2017/09/A128043_2_En_10_Chapter_Equ2.gif)
(10.2)
where λ is the brain/blood partition coefficient; M C and M L are the time-averaged signal intensities in the control and label images, respectively; T 1A is the longitudinal relaxation time of arterial blood; α is the labeling efficiency; S PD is the signal intensity of a proton density-weighted image; τ is the label duration; and PLD is the post-labeling delay. TI and TI1 are the previously defined parameters of QUIPSSII PASL. The factor 6000 converts the CBF units from mL/g/s to mL/(100 g)/min.
In principle, to scale the ASL difference signal to absolute CBF units, the signal intensity of the fully relaxed blood is needed. While different methods have been proposed to estimate this value [51–53], a voxel-wise scaling factor obtained from a separately acquired proton density image with a long TR (>5 s) is most commonly used [41]. The signal intensity of blood is then obtained dividing this image by the partition coefficient λ. Note that, although this coefficient could vary across different tissue types, a brain averaged value is used in practical implementations. Typical values are reported in Table 10.1, together with recommended imaging/labeling parameters [41].
Table 10.1
Recommended ASL parameters for clinical studies at 3 T
pCASL labeling duration (τ) | 1800 ms |
---|---|
pCASL post-labeling delay (PLD) | 2000 ms |
pCASL labeling efficiency (α) | 0.85 |
PASL TI1 | 800 ms |
PASL TI | 2000 ms |
PASL labeling efficiency (α) | 0.98 |
Spatial resolution | 3.5 mm × 3.5 mm × 6 mm |
Scan time | 4 min |
Blood-brain partition coefficient (λ) | 0.9 mL/g |
T1 of arterial blood(T1A) | 1650 ms |
10.6 CBF Quantification in Clinical Studies
While the model at the basis of equations ((10.1) and ((10.2) is simplified, it is considered robust enough to be used as a good compromise in a clinical setting.
A critical sequence parameter for clinical populations is the time delay inserted between labeling and image acquisition that should be long enough to satisfy the first assumption of the CBF quantification model. As explained earlier, this delay is used to allow labeled arterial blood to reach the capillaries. A longer PLD/TI generally reduces the sensitivity of perfusion quantification to uncertainties regarding the precise arrival timing of the labeled blood at different voxels [30, 41]. Note that arterial arrival times may be markedly increased in the diseased brain, suggesting the use of long PLD/TI in clinical populations. However, it should also be considered that longer delays lead to a reduced signal-to-noise ratio, because the magnetic label decays with blood T1 which is comparable with typical PLD values. The choice of PLD is therefore a compromise between acceptable SNR and complete label delivery to ensure that the ASL signal will accurately reflect CBF.
In this regard, ASL perfusion measurements at 3 T are preferred over those at lower fields. Indeed, blood T1 at 3.0 T (1.6–1.8 s) is longer than blood T1 at 1.5 T (1.2–1.4 s), resulting in a better SNR because the label decays more slowly. This also allows the use of longer post-labeling delays to reduce sensitivity to arterial arrival time differences. Furthermore, the increased general SNR at high field can be exploited to decrease scanning time and to acquire higher-resolution images.
As an example of a quantitative CBF map obtained with ASL, Fig. 10.5 shows the results obtained in a recent study investigating the correlation of brain iron accumulation with the severity of vascular damage and cerebral perfusion in Alzheimer disease patients [54].
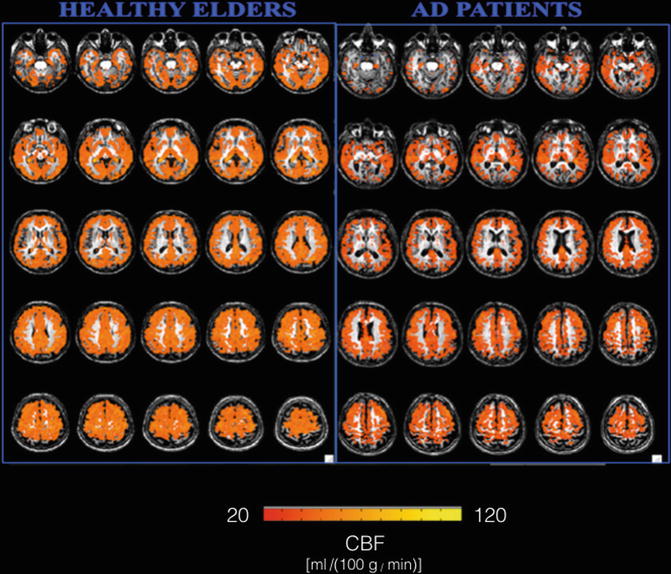
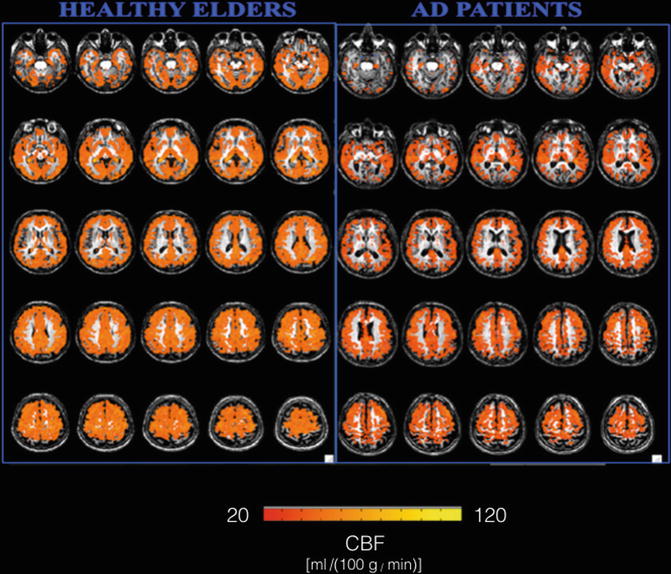
Fig. 10.5
Quantitative CBF maps showing perfusion levels in a group of AD patients compared to normal elderly
Methods based on single PLD/TI ASL imaging can provide a rapid and robust measure of CBF that can be made relatively insensitive to ATT with an optimal choice of sequence parameters. However, these methods do not provide measures of ATT or estimates of errors introduced into the CBF measurement by abnormally long ATT values. These aspects are particularly important in some clinical populations, such as patients with steno-occlusive diseases. In these cases, ASL approaches with multiple PLD/TI can be used together with more sophisticated models to fit the data, yielding both CBF and ATT estimates [55–59]. However, these multiple TI/PLD methods are more complex, requiring more measurements and processing, and are therefore not widespread in the standard ASL clinical setting.
In other advanced applications of ASL, labeling is performed on specific arterial vessels for studies requiring regional perfusion territory imaging [60, 61]. Selective labeling may be useful in patients with high-grade or complete vascular occlusion to guide the choice between angioplasty and stenting, to evaluate cerebrovascular bypass grafts, or to estimate the likelihood of an embolic or atherothrombotic source in a patient with multiple bright regions on diffusion-weighted imaging. For example, Hendrikse et al., selecting labeling on the basis of a previous MR angiogram, showed excellent visualization of perfusion territories corresponding to right carotid, left carotid, and posterior circulation [62–64]. It is important to note that the only way to obtain such information previously was invasive catheter angiography. These data may then be helpful to gain a better understanding of vascular dynamics in patients at high risk of stroke or being considered for surgical procedures [65].
10.7 Applications of ASL in fMRI
While BOLD fMRI [66–68] has evolved into a powerful tool to study brain function, its limitations as a quantitative technique are well known. Indeed, the BOLD response stems from a complex interplay of changes in CBF, cerebral blood volume (CBV), and oxygen metabolism (CMRO2), and therefore the magnitude of the BOLD signal change is not a direct measure of neural activity [69–72]. This limitation is a major drawback when the research question requires a comparison of fMRI data across groups that may differ in both neural activity and neurovascular coupling [73, 74].
A solution to this problem has been to combine BOLD and ASL imaging within a biophysical model of the BOLD effect in order to disentangle the different physiological variables (e.g., CBF, CMRO2) that link neuronal signaling to changes in MR signal decay via blood deoxyhemoglobin content [75–78]. This approach, termed calibrated BOLD imaging, is very attractive because it offers a noninvasive quantitative measurement of cerebral physiology that can be used, e.g., to characterize long-term changes in brain oxygen metabolism in studies of brain diseases and treatment interventions.
Standard BOLD fMRI based on gradient-echo imaging also suffers from low spatial specificity due to a significant contribution of downside large venous vessels to the functional signal. In addition, the macrovascular contribution introduces a significant nonlinearity in the BOLD response [79]. Although spin-echo imaging was shown to reduce the macrovascular contribution and the corresponding nonlinear effects on the BOLD signal [80–82], the increase in spatial specificity to the capillary bed with this technique was considered significant only at high fields (>7 T) [83, 84]. In this regard, despite a lower sensitivity with respect to standard BOLD, the fMRI technique based on ASL has proved to be a suitable tool for mapping neuronal activity changes induced by motor/cognitive tasks or sensory stimulation [85–87]. In particular, ASL showed higher intrasubject reproducibility and a more accurate spatial localization of neuronal activity than BOLD fMRI even at 3 T due to a lower contribution of macroscopic veins to the functional signal [86].
Conclusions
Arterial spin labeling is a MRI technique that allows a noninvasive quantification of cerebral blood flow, which is an important physiological parameter in many brain disorders. The technique has been initially validated against other methods that use exogenous contrast agents, such as PET. In the past two decades, ASL has evolved in a powerful tool capable to obtain high-quality whole-brain perfusion images in a few minutes of scanning. With recent technical advances, ASL implementations are now commercially available on all major MRI platforms, offering an attractive methodology to study brain perfusion and function in clinical populations.
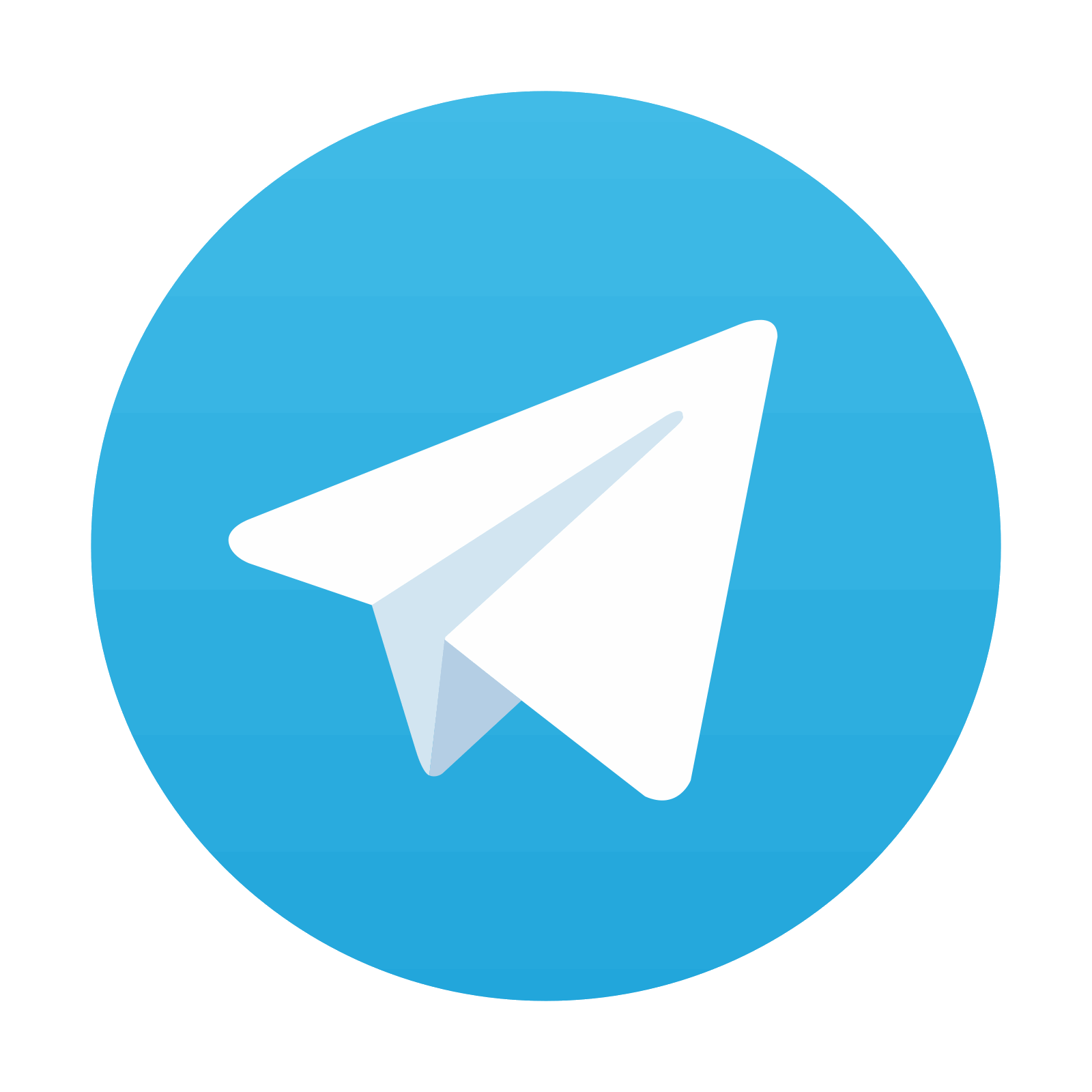
Stay updated, free articles. Join our Telegram channel
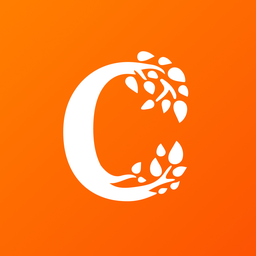
Full access? Get Clinical Tree
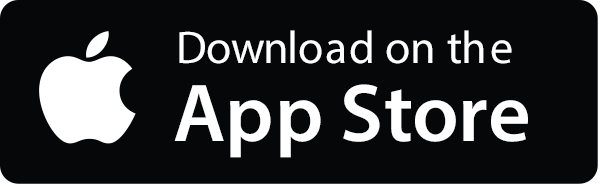
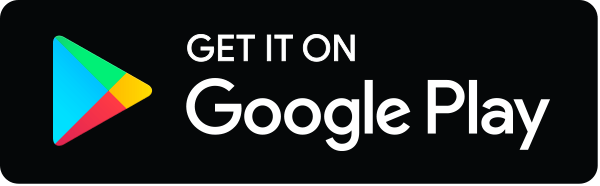