Chapter 11 Brachytherapy
Introduction
• A short period of time which may be measured in minutes as in the case of temporary implants
• A long period, over the effective life-time of the radionuclide during the decay phase, in the case of permanent implants.
Radionuclides in brachytherapy
Gamma emitters
The ideal radionuclide should have the following properties:
Absorbed dose
The special unit for kerma is the gray (Gy) and the relationship between kerma and dose rate, Dr is:
Gamma emitters
Iridium-192
Iridium-192 with a mean gamma energy of 0.370 MeV and half-life of 74 days is now being widely used, taking advantage of its high specific activity and the properties of a flexible wire, in which form it has many advantages over traditional radium or caesium needles. It has been in use since the late 1950s, first in the form of seeds, by Henschke, and then a few years later in the form of wire and hair-pins at the Institute Gustave Roussy in Paris. Coils of thin wire (0.3 mm diameter) can be cut to convenient lengths and inserted into flexible nylon tubes or rigid hollow afterloading needles similar to hypodermic needles, which have been previously implanted into tumours (see Figure 11.1A and B). The active iridium–platinum core is 0.1 mm in diameter and contained within a sheath of platinum 0.1 mm thick. This sheath is adequate to filter out most of the beta-rays produced as a result of the decay process. Beta emmissions are predominantly at energies of 0.530 MeV and 0.670 MeV. Thicker wires, 0.6 mm in diameter, in the form of hairpins (see Figure 11.1C and D) can also be inserted directly into tumours through suitable introducers. In the USA, iridium is available in the form of seeds sealed in thin nylon coated ribbon. Although iridium in the form mentioned here is generally used for low dose rate treatments, it can also be used as a high activity source for HDR systems (see Figure 11.2A and B).
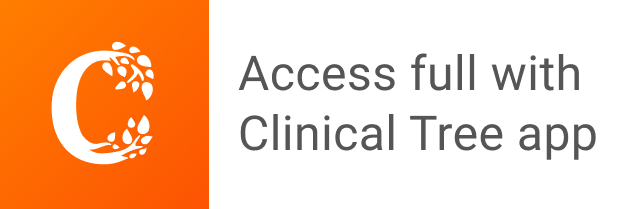