Fig. 1
Reduction of neointima formation due to irradiation: Cross-sections of rabbit carotid arteries at 4 weeks after denudation trauma (magnification × 10). Control artery (left) demonstrates intense neoinitimal proliferation. Following endovascular irradiation from a Re-188-filled balloon catheter (30 Gy at the balloon surface) the proliferation is almost completely inhibited (reprinted with permission from (Kotzerke et al. 2000)
Restenosis is the major drawback of percutaneous coronary intervention (PCI) and occurs even after stenting within 6 months in approximately 30 % of all cases (Califf et al. 1991). Various mechanisms for restenosis have been proposed: migration of myocytes from the media to the intima, forming the neointima (Austin et al. 1985); damage of the adventitia resulting in proliferation of adventitial fibroblasts and migration of these mesenchymal cells to the media and neointima (Wilcox et al. 1996); organization of mural thrombus, resulting in a local hypertrophic scar (Schwartz et al. 1992); and release of cytokines and growth factors, e.g., by monocytes/macrophages which mediate the cellular migration and proliferation (Libby et al. 1992; Rubin et al. 1998). Clinical research aimed at overcoming restenosis has included the administration of mechanical and pharmacological approaches such as atherectomy, rotablation, laser ablation, and drugs, including heparin and irradiation as well as drug-eluting stents.
Restenosis is an important economic issue and the potential cost benefit of restenosis reducing techniques can be estimated in a similar approach performed when comparing coronary stenting and balloon angioplasty as well as PCI and bypass surgery (Cohen et al. 1994; Fischman et al. 1994; Kupersmith et al. 1994, 1995; Reeder et al. 1984). Assuming the restenosis rates of 33 % followed by bypass surgery for the first, second, and third restenosis in 10, 50, and 100 %, respectively, repeated PCI procedure and bypass surgery would be necessary in 35.2 and 12.8 per 100 initial stenoses, respectively (Kotzerke et al. 2000). Assuming the costs of PCI and of bypass surgery to be 3.250€ and 16.000€, respectively, the current cost of treatment of restenosis is 3.200€ per initial stenosis (Kotzerke et al. 2000). The assumption that any approach can reduce restenosis rate by 50 %, repeated PCI procedures, and bypass surgery would be necessary only in 16.2 and 3.5 per 100 initial stenosis, respectively. This would reduce the cost of restenosis treatment from 3.200€ to 1.100€ per initial stenosis. The magnitude of this health problem becomes apparent when one recognizes that stents are reportedly used in as many as three-quarters of the approximately 1 million PCI procedures performed annually in the United States with increasing frequency (Sapirstein et al. 2001).
2 Radiation Sources
Gamma-emitters are characterized by deep tissue penetration and delivery of almost the same dose to all vessel layers. However, radiation protection of the personal and the environment needs considerable care. With the available γ-sources intracoronary dwelling times of approximately 30 min are required (Table 1). Beta-emitters are characterized by high energy delivery but a low tissue penetration which simplifies radiation protection but complicates to achieve a homogeneous dose distribution without centering of the irradiation source. A balloon catheter filled with liquid β-emitter has the advantage of homogeneous dose delivery due to the self-centering irradiation source; however, its application occludes temporarily the coronary blood flow (Table 1) (Amols et al. 1996a, b; Weinberger 1999).
Table 1
Radionuclides used for vascular brachytherapy
Isotope | Emission | Half-life | Energy maximum | Energy average | Activity required |
---|---|---|---|---|---|
– | – | – | [MeV] | [MeV] | [GBq] |
– | – | – | – | – | – |
Ir-192 | Gamma | 74 days | 0.67 | 0.18 | 18.5 |
Sr/Y-90 | Beta | 29 years | 2.28 | 0.93 | 3.7 |
Y-90 | Beta | 64 h | 2.28 | 0.93 | 3.7 |
P-32 | Beta | 14 days | 1.71 | 0.69 | 3.7 |
Re-186 | Beta | 90 h | 1.08 | 0.38 | 11.1 |
Re-188 | Beta | 17 h | 2.12 | 0.77 | 3.7 |
Xe-133 | Beta | 5.2 days | 0.35 | 0.10 | 11.1 |
Sm-153 | Beta | 47 h | 0.81 | 0.22 | 3.7 |
Ga-68 | Beta+ | 68 min | 1.89 | 0.83 | 3.7 |
Cu-62 | Beta+ | 9.7 min | 2.93 | 1.30 | 1.5 |
Ho-166 | Beta | 27 h | 1.85 | 0.67 | 7.5 |
2.1 Beta-Versus Gamma-Emitters
If radiation energy is delivered to a dividing cell, its effects are independent of the source used (Teirstein 1998). That is, cell division should be equally inhibited by gamma and beta energy as long as the energy is brought to the intended target. Gamma rays penetrate human tissues deeply and are not shielded by stents. This makes gamma energy ideal for treating large vessels by a line source and for the treatment of in-stent restenoses. However, there are disadvantages in terms of radiation protection of the patient and personal. A heavy lead shield several centimeters thick is required for effective attenuation of the photons. Furthermore, in the catheterization laboratory, all “non-essential” personal have to leave during the irradiation procedure. The use of lower activity circumvents some of these problems, but irradiation times then have to be extended to achieve prescribed doses. In contrast, beta sources emit electrons with low penetration and high local energy deposition which can easily be shielded even with plastic. Prescribed dose can be achieved within 5–8 min depending on specific volume (Table 2). Tissue penetration is restricted to a few millimeters and use of metallic stents or thick plaque material might restrict effective energy deposition (Amols et al. 1998). Delivery of beta energy using a line source will probably not provide adequate treatment of large-diameter vessels (>4 mm) and will require centring devices (Table 3). However, a meta-analysis of randomized controlled trials of intracoronary gamma- and beta-irradiation therapy for in-stent restenosis demonstrated effectiveness for both radiation sources (Uchida et al. 2006).
Table 2
Variation in radial radiation dose depending on balloon dimension, calculated using the point kernel function. Reference dimension is 3.0 × 20 mm (reprinted with permission from (Kotzerke et al. 2000)
Length [mm] | 10 | 20 | 30 | 40 | 50 |
---|---|---|---|---|---|
Diameter | – | – | – | – | – |
2.0 | 0.67 | 0.67 | 0.67 | 0.67 | 0.67 |
2.5 | 0.84 | 0.85 | 0.85 | 0.85 | 0.85 |
3.0 | 0.99 | 1.00 | 1.00 | 1.00 | 1.00 |
3.5 | 1.12 | 1.12 | 1.13 | 1.12 | 1.12 |
4.0 | 1.22 | 1.23 | 1.23 | 1.23 | 1.23 |
5.0 | 1.37 | 1.39 | 1.39 | 1.39 | 1.39 |
6.0 | 1.48 | 1.49 | 1.49 | 1.49 | 1.49 |
Table 3
Dose gradients with beta (Y-90) versus gamma (Ir-192) sources delivering a dose of 8 Gy at 2 mm depth (adopted from (Waksman 1998))
– | Yttrium-90 [Gy] | Iridium-192 [Gy] |
---|---|---|
– | – | – |
Dose at Lumen | 53.0 | 23.0 |
Dose at 1 mm | 16.0 | 12.0 |
Dose at 2 mm | 8.0 | 8.0 |
Dose at 3 mm | 2.7 | 6.3 |
2.2 Balloon Versus Wire
A gamma source for endovascular irradiation will always be a line source because the deep penetration of the energy obviates the need for an exact geometry. However, when using beta sources the limited penetration makes careful centring essential for homogeneous dose delivery to the vessel wall. A number of systems have been devised to deliver intravascular brachytherapy from removable beta sources including yttrium-90 wire sources (Schneider, Bükach, Switzerland), encapsulated Sr-90/Y-90 seeds (Novoste, Norcross, Ga., USA), and phosphorus-32 seeds (Guidant, Santa Clara, Calif., USA). However, only some of these catheter-based systems had centering capabilities, such as segmented or helical balloons (Popowski et al. 1995). A comparison of a centred P-32 source wire system with a noncentered Sr-90/Y-90 brachytherapy system for intracoronary β-radiation following PCI of diffuse in-stent restenosis yielded a significant superiority of the centered source (Haase et al. 2005). In contrast to a radioactive wire, a beta-emitting radioisotope-filled balloon provides a radiation field that conforms to the vessel geometry in an optimal fashion regarding the vessel lumen (Amols et al. 1996a; Weinberger 1998). Centering of the radiation delivery balloon occurs during inflation even in vessels bend. The radiation dose to the vessel wall is uniform and can be prescribed easily based on the specific volume of the beta-emitter and the balloon’s dimensions (Table 2). Larger vessels can be irradiated using a more voluminous balloon catheter, resulting in a shorter dwell time in comparison with radioactive beta emitting wires, which necessitate much longer irradiation times. A disadvantage of the balloon technique is the occlusion of the vessel, which will be tolerated only for a limited time in the case of the coronary arteries. However, the balloon can be easily deflated and irradiation can be fractionated according to the clinical symptoms (Hoher et al. 2000, 2003). After re-opening of an occluded vessel, the collateralization will prevent pain even during time-consuming irradiation procedures. The main disadvantage is the risk of balloon rupture or leakage with resultant patient contamination (Hausleiter et al. 2000). The prime potential candidates for the liquid-filled balloon are high-energy beta-emitters such as Y-90, P-32, Re-186, Re-188, Ga-68, Ho-166, or Sm-153. However, bone- and bone marrow-seeking isotopes such as Y-90 and P-32 cannot be used because of the high radiation absorbed dose that would occur in the event of balloon rupture. Compared with Re-186, Re-188 has the advantages of higher beta energy and availability from a generator and it is therefore the most promising candidate. The “hot balloon” filled with Xe-133 might be an alternative and carries only a low risk of incorporation in the event of balloon leakage because it would be exhaled. However, it is much more expensive than Re-188. Ga-68, a positron emitter, has also been proposed (Stoll et al. 2001). However, the advantage of the short half-life of 68 min in the unlikely event of leakage is an important disadvantage for routine use: generator cannot be eluted in advance but on demand to obtain the highest specific volume and the shortest irradiation time. Moreover, radiation protection of the stuff is much more difficult to realize because of the 511 keV gamma emission. In clinical routine, Re-188 was and is used most.
2.3 Radioactive Stents
Hehrlein et al. introduced activated Palmaz-Schatz stents which were placed in a cyclotron and bombarded with deuterons (Hehrlein et al. 1995). Later, proton bombardment was used to reduce the portion of high-energy gamma radiation emitted by the stents. A mixture of radioactive isotopes was created (e.g. cobalt-55, -56, and -57, nickel-57 and iron-57) with different energies and half-lives. This stent delivered most of the energy within 5 days and 15–20 % within 20 and 260 days.
Another method was based on the selective implantation of a single radioisotope into stents (Hehrlein et al. 1996). The activity of the emitting stents used in clinical trials ranged from 19 to 222 kBq. The dosimetry of a P-32 stent has been previously described in detail by Janicki et al. (Janicki et al. 1997): for a 37 kBq 15-mm-long P-32 stent at a distance of 0.1 mm dose values of 25 Gy are delivered at the strut wires (peaks) and 8 Gy between the wires (valleys) voer on half-life. The nonuniformity of dosing, which reflects the stent geometry, decreases at distances of 1–2 mm from the surface (Carter and Laird 1996). The actual dose distribution will also be affected by variations in atherosclerotic plaque morphology and the symmetry of stent expansion. Another development was the coating of a stent with Re-188 or Re-188 directly before implantation (Hafeli et al. 1998). This method allows any used stainless steel or tantalum stent to be coated with radioactive electroplating solutions, one of which contains radioactive rhenium. The overall processing time is only 15 min.
Unfortunately, initial clinical trials using the P-32 stent demonstrated restenosis rates of approximately 50 %, largely due to intimal proliferation at the stent edges, so-called “candy wrapper stenosis” (Albiero et al. 2000; Serruys and Kay 2000). These clinical failures have terminated further studies and closed the use of radioactive stents (Teirstein and Kuntz 2001).
3 Rhenium-188: Production, Properties, Dosimetry, and Safety
Carrier-free Re-188 can be obtained from the W-188/Re-188-generator by elution with saline (Knapp et al. 1997). Coupling the parent radioisotope to alumina, and loading on a column, allows production of a W-188/Re-188-generator (Callahan et al. 1989). The parent W-188 has a half-life of 69 days, while the daughter isotope Re-188 possesses a half-life of 17 h. Depending on starting activity, the generator system can be used for up to 6 months. The specific volume of the radiotracer can be increased by anion exchange columns to as high as 20 GBq/ml and a semi-automated system for elution and concentration has been developped (Oh et al. 2003; Wunderlich et al. 2008). Re-188 is an ideal candidate regarding the long half-life of 69 days of the parent W-188 and the properties of the daughter Re-188: short half-life of 17 h, high-energy beta particles (Eβmax = 2.12 MeV, average energy 764 keV) for therapeutic use and gamma emission of 155 keV (15 % intensity) for imaging purposes. The latter one also permits simple contamination control of the patient and his surroundings.
Radiation absorbed dose from a balloon catheter has been measured by means of thermoluminescent dosimetry (TLD) and compared to calculations using point kernel function of Re-188 (Kotzerke et al. 1998b). A very good correlation between these methods was demonstrated for the absolute radiation absorbed dose which showed a rapid reduction of 50 % within 0.5 mm. Assuming a specific volume of 3.7 GBq/ml at the surface of a typical balloon catheter (3.0 x 20 mm, 135 μl volume) and at 0.5 mm distance (i.e., 2 mm distance from the centre of the balloon), doses of 7.8 and 3.9 Gy/min could be achieved, respectively (Fig. 2) (Kotzerke et al. 1998b). It was calculated that the filling pressure of the balloon catheter is not critical for variation of the balloon volume and varies by only 1 % per atmosphere filling pressure (Kotzerke et al. 1998b). Using the point kernel function, the radial radiation dose of other balloon dimensions was calculated (Table 2). Similar Tables were calculated for various radionuclides including P-32, Y-90, and Re-188 (Fox 1997; Fox and Henson 1999; Fox et al. 1999).
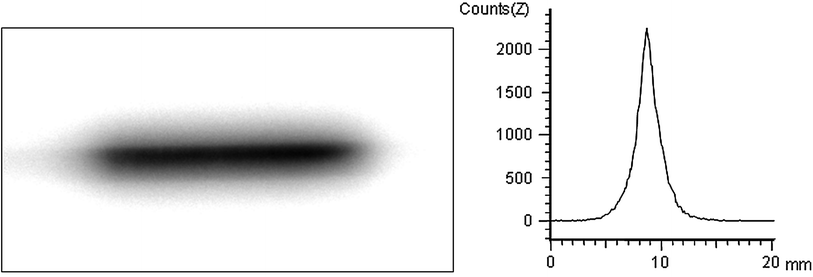
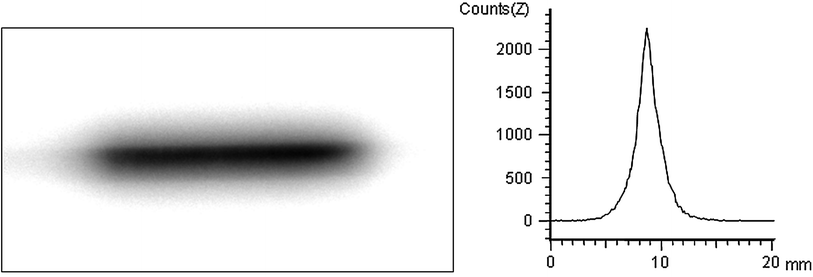
Fig. 2
Re-188 filled balloon catheter: Energy distribution from a Re-188-filled balloon catheter as visualized by a Phosphor imager (PosphorImager SI, Molecular Dynamics GmbH, Krefeld, Germany). The transaxial activity histogram demonstrates the low penetration of the beta particles and the steep dose drop off (reprinted with permission from (Kotzerke et al. 2000))
The radiation exposure due to intravascular application of a radionuclide depends on physical properties and its biological pharmacokinetics. Perrhenate will behave in the human body like pertechnetate with accumulation in the thyroid and the gastric mucosa as well as urinary excretion. To minimize the radiation exposure in the hazardous event of balloon rupture, chelation of Re-188 for increased renal elimination was proposed (Cho et al. 2004; Lee et al. 2000; Lin et al. 2000). The total body absorbed dose would be reduced to 10 % by the chelation. Another proposal has been the use of perchlorate following an unexpected release of Re-188 into the blood. Kotzerke et al. demonstrated that perchlorate will discharge perrhenate from the thyroid and stomach and will reduce the effective dose to 0.16 mSv/MBq (i.e., 38 % compared to the unblocked state) (Kotzerke et al. 1998a). Assuming a balloon catheter of 3.0 x 20 mm filled with 135 μl Re-188 in a specific volume of 1.85 GBq/ml, 259 MBq Re-188 would be released in the event of rupture. This acitivity would result in an effective dose equivalent of 109 mSv, which could be reduced to 41 mSv by oral administration of perchlorate. Forced diuresis might decrease the radiation absorbed dose even further.
A single balloon rupture has been reported ever (Hausleiter et al. 2000). In the largest trial of intracoronary irradiation using Re-188 filled balloon catheter no patient contamination has been observed nor acute or chronic injuries of the hands of the applicating physicians (Hoher et al. 2003). The very low probability of balloon rupture combined with the acceptable radiation absorbed dose from Re-188 and the effective discharge from the critical organs achieved by subsequent administration of perchlorate should render the chelating procedure unnecessary. This would save time, money, reduce radiation exposure to the technician and guarantee a maximum specific volume of perrhenate, thereby minimizing the irradiation time.
4 Recommendations and Standardization for Vascular Brachytherapy
Neither the exact absolute dose needed for successful vascular radiotherapy nor the optimal spatial and temporal distribution of dose inside the vessel lumen or wall is known (Quast et al. 2002). Many different irradiation techniques have been employed in experimental and clinical studies with good success. However, in most studies the doses delivered were prescribed, described, and reported poorly or inconsistently (Quast et al. 2002), complicating a meta-analysis. The American Association of Medical Physicist (AAMP) has provided a set of recommendations in their report on intravascular brachytherapy physics, but these recommendations are applicable only for radioactive wires and cannot completely transferred to liquid-filled balloon catheters (Nath et al. 1999). For example, one of the recommendations was specification of dose to a reference point 2 mm distant from the axis and the centre of a catheter-based system. However, in the case of a radioisotope-filled balloon the vessel surface, which is independent of the vessel diameter, represents a better reference point. Another important paper has dealt with the measurement of the beta energy. The National Institute of Standards and Technology (NIST) published radioactivity standards for beta-emitting radionuclides used in intravascular brachytherapy concerning standard reference material, calibration for source manufactures and calibration factors for commercial instruments (Coursey et al. 1998). Uncertainties in the activity calibration for P-32, Sr–90/Y–90, and Re-188 are in the order ±0.5 %.
However, taken into consideration the real conditions of the irradiation procedure these recommendations seem too rigide. Irradiation is performed during heart beating which might cause also a movement of the irradiation source. Coronary vessels have curvatures which may cause huge differences in regional absorbed irradiation dose (Yue et al. 2004). The metal of the stents may attenuate the absorbed irradiation dose (about 10 %)—should we adjust for that or not? What about overlap in long irradiation fields and re-irradiation? Even in the application of a liquid radioisotope filled balloon catheter which blocks the vessel and provides optimal contact of the irradiation source with the target arterial segment—the vessel tapers off which may cause an inhomogeneous irradiation absorbed dose along the balloon.
5 Regulations
Besides the recommendations of the AAMP (Nath et al. 1999) several national and international (european) recommendations have been launched. The German Society of Cardiology published a position paper on intracoronary brachytherapy (Dietz et al. 2001). The Endovascular Groupe Européen de Curiethérapie/European Society for Therapeutic Radiology and Oncology Working Group published recommendations on prescribing, recording in endovascular brachytherapy including quality assurance, equipment, personnel, and education (Potter et al. 2001). The recommendations of the AAMP were adopted in national legal force (Quast et al. 2002). The German Radiation Protection Commission developed recommendations to perform intracoronary irradiation for implementation in the german “Richtlinie Strahlenschutz in der Medizin” (Herrmann 2001). A further recommendation from the Vienna group adressed the determination of planning target length to avoid geographic miss (Schmid et al. 2004). The task force for percutaneous coronary interventions of the European Society of Cardiology recommended brachytherapy to treat in-stent restenosis in native coronary arteries: IA; for brachytherapy to treat in-stent restenosis in saphenous venous bypass grafts: IB. The Food and Drug Administration (FDA) approved two devices for coronary artery brachytherapy because local intracoronary irradiation showed evidence to be an effective intervention for reducing the recurrence of obstruction after successful treatment of in-stent restenosis (Sapirstein et al. 2001). However, meanwhile both devices were withdrawn from the market.
6 Initial Experience of Radiotherapy in Animal Models of Restenosis
Wiedermann et al. and Waksman et al. were the first to demonstrate significant reduction in intimal proliferation using radiotherapy in the swine model of restenosis (Waksman et al. 1995; Wiedermann et al. 1994). Wiedermann et al. used a swine balloon overstrech injury model of coronary injury to test iridium-192 (Ir-192, a gamma-emitter), delivered 20 Gy over a 30–45 min dwell time. Morphometric analysis at 30 days demonstrated a maximal neointimal area of 0.84 ± 0.60 mm2 in control animals compared with only 0.24 ± 0.13 mm2 in treated animal (P = 0.001). At 6 months follow-up, these differences were 1.59 ± 0.78 versus 0.46 ± 0.35 mm2 (P ≤ 0.001) (Wiedermann et al. 1995). Later, Waksman et al. provided insight into the target of vascular radiotherapy and its mechanism of action (Waksman et al. 1997). Balloon injury was performed on swine coronary arteries, followed immediately by either Sr-90/Y-90 or Ir-192 sources designed to deliver 14 or 28 Gy at a depth of 2 mm from the source. Animals were sacrificed at 3, 7, or 14 days. Bromodeoxyurdine was administered 24 h before scarifying to label proliferating cells. On day 3, cellular proliferation was significantly reduced in both the adventitia and the media of treated vessels compared with controls. At 2 weeks postinjury, there were fewer α-actin-positive myofibroblasts in the adventitia of treated compared with control animals, and morphometric analysis indicated the vessel perimeter of treated vessels was significantly larger than controls. Apoptosis was estimated by terminal transferase dUTP-biotin nick-end labeling (TUNEL) at 3 and 7 days after injury. No differences in TUNEL-labeled cells were found between treated and control vessels. These studies suggest that intracoronary radiation primarily inhibits cellular proliferation in both the media and adventitia and suggests a mechanism other than apoptosis. They also suggest a favorable effect on late remodeling probably by preventing adventitial fibrosis at the injury site. Numerous other investigators have demonstrated the efficacy of both, gamma- and beta-radiation in various animal models of restenosis including Re-188 filled balloon (Wohlfrom et al. 2001). All studies were performed as balloon injury which is a model of de novo stenosis. In-stent restenosis were not addressed in animal studies (Teirstein and Kuntz 2001).
7 Clinical Trials
7.1 In-Stent Restenosis
Seven double-blind, randomized clinical trials have investigated the efficacy of intracoronary brachytherapy in patients with in-stent restenosis (Fig. 3) (Grise et al. 2002; Leon et al. 2001; Popma et al. 2002; Raizner et al. 2000; Teirstein et al. 1997, 2000; Waksman et al. 2000, 2002b, 2002a). The Scripps Coronary Radiation to Inhibit Proliferation Post-Stenting (SCRIPPS) study was the first double-blind, randomized study that investigated the effect of intracoronary brachytherapy on in-stent restenosis (Teirstein et al. 1997). 55 patients were randomized to intracoronary brachytherapy with iridium-192 or placebo. At 3 year follow up, the incidence of death, myocardial infarction, and target vessel revascularization was 23 % in the brachytherapy group versus 58 % in the placebo group (P = 0.01) (Teirstein et al. 2000). At 5-year follow-up, the incidence of death, myocardial infarction, and target vessel revascularization was 38 % in the brachytherapy group versus 65 % in the placebo group (P = 0.02) (Grise et al. 2002).
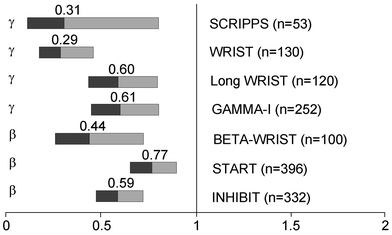
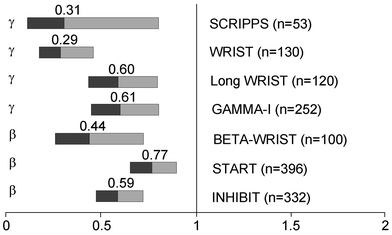
Fig. 3
Summary of benfit of intracoronary brachytherapy versus conventional treatment for restenosis prevention in main randomized controlled trials (adopted from (Sabate 2009))
The Washington Radiation for In-Stent Restenosis Trial (WRIST) was a double-blind, randomized study that investigated the use of catheter-based low-dose gamma radiation (iridium-192) in 130 patients with in-stent restenosis (Waksman et al. 2000). At 6-month follow-up, the incidence of angiographic restenosis was 19 % in the brachytherapy group versus 58 % in the placebo group (P = 0.001). At 6-month follow-up, the incidence of death, myocardial infarction, and target vessel revascularization was 29 % in the brachytherapy group and 68 % in the placebo group (P = 0.001).
The Proliferation Reduction with Vascular Energy Trial (PREVENT) was a double-blind, randomized, sham-controlled study of intracoronary brachytherapy using a beta source of brachytherapy (phosphorus-32) in 105 patients with either de novo lesions or restenotic lesions (Raizner et al. 2000). At 6-month follow-up, the incidence of restenosis was 8 % in the brachytherapy group versus 39 % in the control group (P = 0.0012). At 6-month follow-up, the incidence of death, myocardial infarction, and target vessel revascularization was 26 % in the brachytherapy group versus 32 % in the control group (P not significant).
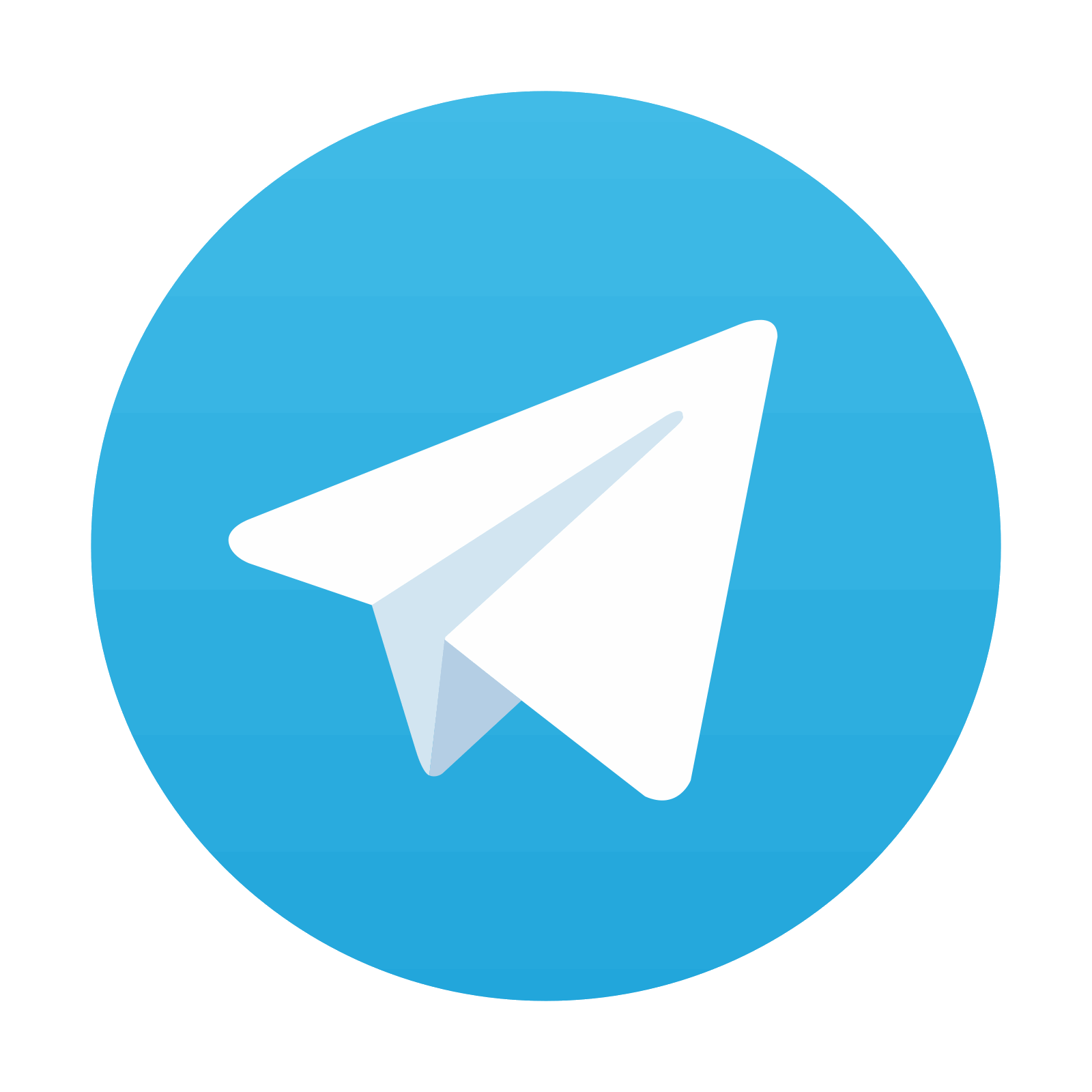
Stay updated, free articles. Join our Telegram channel
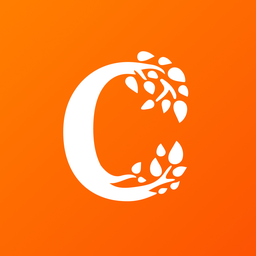
Full access? Get Clinical Tree
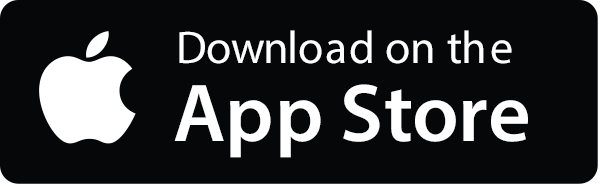
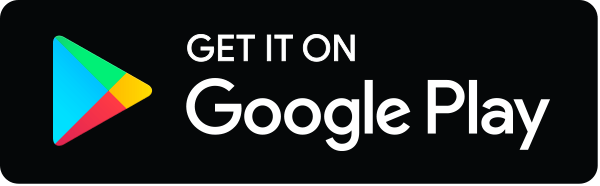