Fig. 8.1
fMRI-guided TMS neuronavigation. Panel (a) shows several color-coded fMRI activity clusters superimposed on a reconstruction of the cortical surface, projected within a transparent mesh of a reconstructed head in Talairach space. Each of these clusters represents an individual fMRI “hotspot,” i.e., strongest task-related activity, of a given individual participant obtained in a separate fMRI measurement. The spatial distribution between these individual fMRI activity clusters accounts for the interindividual variability in the exact structure-function correspondence. Panel (b) shows a snapshot of the Brain Voyager TMS neuronavigation system used to guide TMS coil positioning based on one of these activity clusters of a given participant. The red beam indicates where the magnetic field of TMS is strongest and is navigated in real time to the orange color-coded individual fMRI hotspot of this particular participant. The exact positioning of the TMS coil and thus the target area for the magnetic brain stimulation are therefore individually defined based on the fMRI data obtained in a separate session prior to TMS
Using such neuronavigation systems, TMS coil positioning can become highly accurate, targeting anatomical or functional “hotspots” in individual participants with millimeter precision. This is relevant since, despite the limited spatial resolution of the applied magnetic field, spatial TMS coil shifts in the order of millimeters have been shown to sometimes result in a complete loss of behavioral or cognitive impairment effects (Beckers and Homberg 1992; d’Alfonso et al. 2002). Comparing different localization strategies for TMS-based primary motor cortex mappings in terms of accuracy and efficiency, Sparing and colleagues (2008) found that fMRI-guided stimulation was most precise (accuracy was concluded to be in the millimeter range). Feredoes and colleagues (2007) used fMRI to localize TMS sites for disruption of short-term verbal information retention. Sack et al. (2009) investigated the behavioral impact of right parietal TMS on a number comparison task, when TMS localization was based on (1) individual fMRI-guided TMS neuronavigation, (2) individual MRI-guided TMS neuronavigation, (3) group functional Talairach coordinates, or (4) the 10–20 EEG position P4. They quantified the behavioral effect of each TMS localization approach, calculated the standardized experimental effect sizes, and conducted a statistical power analysis, which revealed that the individual fMRI-guided TMS neuronavigation yielded the strongest behavioral effect size (Sack et al. 2009). This increased effect size of TMS when using (f)MRI-guided coil positioning has also been shown in the context of clinical TMS applications for various psychiatric disorders (Ahdab et al. 2010; De Ridder et al. 2011; Herbsman et al. 2009).
8.1.3.2 Brain Imaging After Brain Stimulation
Certain brain stimulation protocols, such as rTMS or TBS, are capable of modulating neural excitability of a region beyond the TMS stimulation itself. Functional imaging can then be used to investigate these prolonged TMS aftereffects. Imaging the immediate and longer-lasting aftereffects of TMS is paramount for revealing the underlying neurobiological mechanisms that lead to the observed behavioral changes and clinical treatment effects of TMS stimulation.
An elegant example of this approach comes from Hubl and colleagues (2008). Here, the right frontal eye field (FEF) was stimulated outside the MR scanner using continuous theta burst rTMS (TBS). Then fMRI was used to map the TBS-induced effects and assess their temporal persistence across the brain during a saccade task. The results showed a TBS-induced suppression of local BOLD activity that appeared 20–35 min (but not immediately) after stimulation (Hubl et al. 2008). Suppression, albeit weaker, was also evident in more remote regions, including the (pre)supplementary and parietal eye fields. Similarly, Cárdenas-Morales and colleagues (2011) used fMRI for exploring the aftereffects of iTBS over primary motor cortex.
Several studies have used functional imaging to visualize TMS aftereffects in prefrontal cortex (PFC), in order to explore the underlying mechanisms of potential therapeutic applications for depression (Fitzgerald et al. 2007). The implication is that prefrontal rTMS in normal and depressed participants has profound effects on both local and remote brain regions implicated in depression, including bilateral frontal, limbic, and paralimbic areas (Fitzgerald et al. 2007; Kimbrell et al. 1999, 2002; Pogarell et al. 2006, 2007; Speer et al. 2000, 2009; Teneback et al. 1999). Importantly, these rTMS-induced effects appear to be frequency dependent, with low-frequency rTMS leading to bilateral reduction in frontal activation (Fitzgerald et al. 2007).
8.1.3.3 Brain Stimulation During Brain Imaging
While useful, functional imaging after TMS application remains fundamentally limited in elucidating the neuronal effects of TMS. Concurrent TMS and neuroimaging offer a broader range of in vivo information regarding the actual and immediate effects of TMS on cortical activation, both local and remotely. Simultaneous TMS and imaging can thus be used to online track the TMS effects in the brain or probe intracerebral connectivity (Bestmann et al. 2003b, 2004, 2005; Bohning et al. 1999, 2000b; Ruff et al. 2006; Sack et al. 2007). Therefore, even in the absence of overt behavior, TMS during fMRI facilitates the imaging of pathways of activity spreading within and between brain networks. Furthermore, in simultaneous TMS/fMRI, brain stimulation can be applied while concurrently recording changes in brain activity and behavior. This simultaneous approach allows the investigation of the local and remote brain responses at a neurophysiological level. Thus, it can be determined, in vivo, which brain areas—either directly or transsynaptically affected by TMS—underlie the observed TMS-induced behavioral changes during active task execution. However, the simultaneous combination of TMS and functional imaging poses great technical challenges. Therefore, it is routinely used by only few research groups, and the number of simultaneous TMS/fMRI publications is still considerably small (Reithler et al. 2011).
Besides the need for specific hardware (e.g., an MR-compatible TMS system), simultaneous TMS and BOLD fMRI requires appropriate temporal synchronization between MRI acquisition and TMS pulse application. Furthermore, the discharge, and even mere presence, of MR-compatible TMS coils in the bore of the magnet produces artifacts in the echo-planar imaging (EPI) images that need to be resolved before the simultaneous combination of functional imaging and brain stimulation becomes feasible.
Setup, Experimental Procedures, and Artifacts
The use of TMS inside the MR scanner during simultaneous TMS/fMRI studies requires several modifications to TMS hardware, specific TMS/fMRI interleaved experimental designs, and the consideration or removal of several artifacts. Most importantly, the standard TMS coils routinely used outside the MR scanner are not appropriate for simultaneous TMS/fMRI studies. Instead, specific MR-compatible non-ferromagnetic TMS coils are required. MR-compatible TMS coils are characterized by several main modifications: (1) removal of ferromagnetic materials and electronic elements from the coil, (2) strengthened casing to withstand the large forces of the MR scanner without cracking, (3) a connection cable long enough to feed through a wave guide leaving the radiofrequency (RF)-shielded cabin, and (4) removed or modified TMS coil handle to ease positioning within the spatially restricted MR environment. Finally, since frameless stereotaxy is not applicable inside the scanner, TMS coils are often fitted with specific MR markers in order to post hoc identify the position of the coil relative to the simultaneously acquired structural and functional data. The MR signal of these markers on the TMS coil can be used to estimate and reconstruct, by triangulation, the exact position and orientation of the coil inside the scanner.
Although necessary, these TMS coil modifications are by no means sufficient to avoid further technical problems and measurement artifacts during simultaneous TMS/fMRI. One principle problem of combined TMS/fMRI studies is a direct consequence of the standard TMS/fMRI setup described above. In this setup, the MR-compatible TMS coil is connected to the stimulator outside the RF-shielded cabin via a cable running through a wave guide. Therefore, the RF shield of the MR scanner is pierced by the TMS cable, which acts as an antenna transmitting RF noise into the scanner. Special RF noise filters then need to be installed for simultaneous TMS/fMRI studies as an additional hardware component (Fig. 8.2).
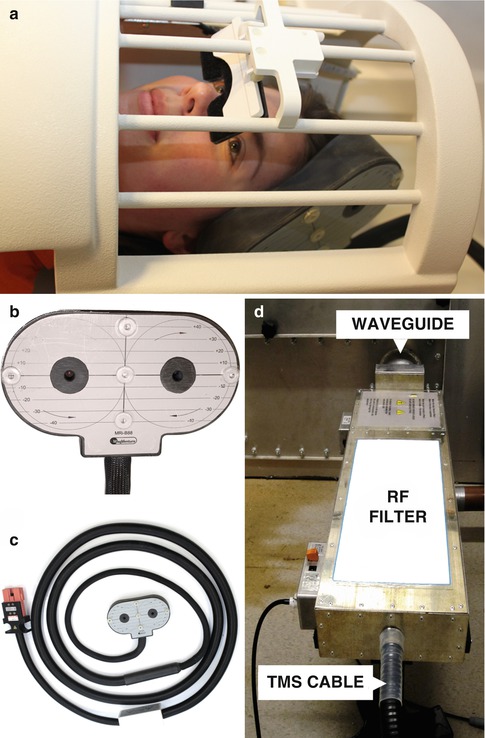
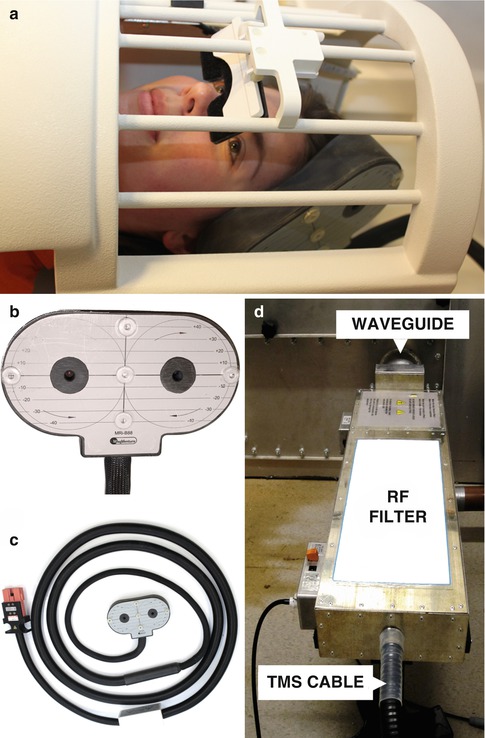
Fig. 8.2
TMS during fMRI. Panel (a) shows a participant inside the MR scanner during simultaneous TMS and fMRI measurements. The participant’s head is fixated within the MR head coil, while an MR-compatible non-ferromagnetic TMS coil is positioned on the scalp and fixated in order to apply noninvasive brain stimulation during functional brain imaging. Panel (b) shows a top view of the MR-compatible non-ferromagnetic TMS coil, which is fitted with five specific MR markers. The MR signal of these markers can be used to estimate and reconstruct, by triangulation, the exact position and orientation of the coil inside the scanner and to thus post hoc identify the position of the coil relative to the simultaneously acquired structural and functional data. Panel (c) depicts the long connection cable of the MR-compatible TMS coil. This cable is used to connect the MR-compatible TMS coil to the stimulator outside the RF-shielded cabin via a wave guide. Panel (d) shows the special RF noise filters that need to be installed for simultaneous TMS/fMRI studies as an additional hardware component. This is necessary because the connecting TMS cable running through the wave guide pierces the RF shield and acts as an antenna transmitting RF noise into the scanner. Therefore, the TMS cable outside the RF cabin is connected to this specific RF noise filter device which connects via the wave guide to the inner RF cabin wall, to then connect to the MR-compatible TMS coil
Despite the installation of an RF filter, the MR image quality is often still decreased in simultaneous TMS and fMRI studies. This is because the mere presence of a TMS coil in the scanner can result in static magnetic field inhomogeneities, which particularly affect EPI scans (commonly used for fMRI). Baudewig and colleagues (2000) systematically investigated the type and extent of the artifacts induced by the TMS coil during MR measurements. The authors revealed that although the anatomical images were unaffected, there were pronounced signal losses and geometric distortions in EPI acquisitions perpendicular to the plane of the coil. However, these artifacts could be markedly reduced by using an EPI orientation parallel to the coil plane. Furthermore, these signal losses and geometric distortions attenuate with increasing distance from the coil and so are restricted to the area very close to the coil. Therefore it is unlikely that functional images of the human cortex are largely affected, given the scalp-cortex distance of >1 cm.
After having addressed the technical challenges discussed above, one can progress to the most important step of applying TMS pulses during actual MR EPI data acquisition. Although, it must be noted that simultaneous or concurrent TMS/fMRI is not possible in the strictest sense. In reality, TMS pulses and MRI acquisitions must be appropriately interleaved in order to avoid the inevitable artifacts produced by the TMS-induced currents, which would otherwise make artifact-free scanning during TMS impossible. Therefore, simultaneously or concurrently combined TMS/fMRI studies, generally refers to interleaved TMS and fMRI measurements, during which the MR sequence must send a trigger signal to the TMS apparatus with every RF pulse excitation. TMS pulses are thus temporally separated from MR imaging. Still, distortions can even occur when pulses are applied up to 100 ms before slice acquisition onset (Bestmann et al. 2003a; Shastri et al. 1999). These lasting artifacts are purportedly related to residual currents in the TMS coil and to currents induced by the vibrations in the TMS coil following a pulse (Shastri et al. 1999). The current standard is, therefore, to leave at least 100 ms between each TMS pulse and any following MR image acquisition. However, with better vibration absorption in the TMS coil, the delay between TMS pulse and MR image acquisition may be reduced considerably.
There are various methods for temporally interleaving TMS and MRI for simultaneous experiments. For example, TMS pulses and MR images can be interleaved by insertion of temporal gaps after each volume (Ruff et al. 2006; Sack et al. 2007). Sack and colleagues (2007) applied bursts of rTMS at ~13.3 Hz over 560 ms at the end of each MR volume. In this study, a delay of 200 ms from the last TMS pulse to the beginning of the next MR volume acquisition protected the subsequent MR acquisition from pulse-related artifacts. Alternatively, TMS pulses can be separated, not by placing them at the end (or beginning) of each volume, but by interleaving them after each slice within one volume (Bestmann et al. 2004, 2005; Bohning et al. 2000a). This method still requires a sufficient delay between TMS pulses and slice acquisition so that subsequent slices are not perturbed. Finally, single slices might also be deliberately perturbed by the TMS pulse and then be identified and replaced, either by interpolation between pre- and post-pulse acquisition of the same slice or by including affected slices as covariates in a general linear model analysis. When employing any of these methods with modified EPI sequences to optimize interleaved TMS/fMRI measurements, it is also recommended to introduce oversampling of the phase-encoding direction of EPI images in order to shift the so-called “ghosting” artifact outside the volume of interest.
One additional problem for simultaneous, or interleaved, TMS/fMRI studies was shown by Weiskopf and colleagues (2009). The authors reported that leakage currents may be generated when switching stimulation intensities. In a phantom measurement, these leakage currents in the TMS coil varied parametrically with the TMS output intensity (its capacitor charge) and induced magnetic field inhomogeneities which led to false-positive fMRI findings. In other words, BOLD signal increased parametrically with TMS intensity in their phantom measurement (Weiskopf et al. 2009). Following this report, a technical solution has been pioneered which introduces a relay in parallel (and diodes in series) with the TMS coil. When the relay is closed, leakage current primarily flows through this relay, rather than the TMS coil. A trigger signal then briefly opens the relay so that a TMS pulse can be applied. However, although these (or similar) solutions are now standard in MR-compatible TMS systems, appropriate test measurements should be run in order to identify any remaining artifacts or false positives due to leakage current.
TMS Has Local and Remote Effects
Generally, all reported studies using concurrent TMS-fMRI show that TMS has task-specific effects on the BOLD signal in the targeted site. This is encouraging, given the widespread assumption that TMS affects excitability/activity in the region directly underneath the coil and that this activity change reflects behavioral effects of TMS (see Reithler et al. 2011, for an exhaustive overview). However, one of the most important additional conclusions from combined TMS and functional imaging studies is that locally applied focal TMS does not exclusively affect neural activity at the stimulation site, but can also be shown to affect remote and interconnected brain regions (Bestmann et al. 2003b; Blankenburg et al. 2008; Bohning et al. 2000a; Denslow et al. 2005; Ruff et al. 2006; Rushworth et al. 2002; Sack et al. 2007). This includes cortical as well as subcortical brain areas, as revealed by early application to the human motor system (Baudewig et al. 2001; Bestmann et al. 2004; Bohning et al. 1999, 2000a). It seems that application of TMS in essence involves inserting energy into a system and that TMS to an isolated neuron population will excite not only that population, but a connected brain area will propagate the inserted energy throughout its anatomical (Boorman et al. 2007) and functional (Sack 2006) network. It is precisely the value of TMS-fMRI that this spread of TMS excitation can be tracked throughout the brain. Bohning et al. (1999) showed that the BOLD signal resulting from TMS correlated to the TMS intensity both in local (targeted) and remote brain areas. Moreover, Bohning and colleagues (2000a) could show that TMS-induced finger movements resulted in BOLD signals throughout the brain that were similar to BOLD signals resulting from voluntary finger tapping. This constituted an early demonstration of the validity of using TMS-fMRI to probe functional/anatomical networks in the brain. Bestmann and colleagues (2004) confirmed this notion, stimulating with high-frequency rTMS the left primary sensorimotor cortex (M1/S1) at supra- and subthreshold intensities (no finger movements induced in the latter condition) and measuring the BOLD signals throughout the brain. A network of distinct cortical and subcortical motor system structures was activated in response to the TMS, again involving the same regions activated by voluntary finger movements. Interestingly, this was the case even for subthreshold stimulation, showing that TMS can probe an anatomical network even in the absence of overt behavioral response, although subthreshold stimulation in the absence of induced muscle contractions mainly led to enhanced BOLD responses in supplementary and premotor cortices and not in the local M1/S1 region that was actually stimulated (see Hanakawa et al. 2009 for similar intensity-dependent remote activation changes based on spTMS). This suggests that the local BOLD effects, directly underneath the coil, may constitute a special case: they depend on actually induced muscle contractions, while remote-connected motor network regions also involved in voluntary movements are activated by M1/S1 TMS even subthreshold (Bestmann et al. 2004; Denslow et al. 2005). Based on modeling work, Esser and colleagues (2005) suggest that TMS locally stimulates both excitatory and inhibitory neural populations (ergo the net activation and thus BOLD is weaker here), but remotely results mainly in excitatory responses which are easier to detect. However, the matter is not settled, given the still ill-defined intricacies of TMS effects on local neural circuits and moreover the connection between such effects and the BOLD signal (Logothetis 2008; Logothetis et al. 2010). Still, the anatomical and functional specificity of the observed remote network effects argues against a nonspecific (water ripple-like) spread of TMS-induced activity. Moreover, the observed networks closely resemble the brain systems involved in natural tasks involving the same regions. For a more elaborate review of these issues, see Reithler and colleagues (2011).
Local and Remote TMS Effects Are State/Task Dependent
Focal TMS can therefore lead to both local and remote neural effects, within anatomically or functionally connected brain regions. However, several combined TMS/fMRI studies have also found that these local and remote network effects are state or task dependent. In other words, the state of the brain at the moment of TMS, as induced by task demands or external sensory stimulation, or even by naturally occurring fluctuations, can influence the local and remote network response to TMS. An excellent example of these state-dependent effects comes from Bestmann and colleagues (2008), who applied TMS over left dorsal premotor cortex (PMd) at two intensities (low vs. high) and two motor states (grip vs. no grip). Participants were stimulated over left PMd either when performing a handgrip task with their left hand or during rest (nogrip). The authors revealed a significant crossover interaction between motor state and TMS intensity over left PMd, arising in right M1 and right PMd. TMS over left PMd during rest led to an activation decrease in right PMd and M1 of the contralateral hemisphere. This contralateral decrease following TMS has been observed in most (Bestmann et al. 2004; Kemna and Gembris 2003), but not all, simultaneous TMS/fMRI studies over the motor cortex (Bohning et al. 2000a; Hanakawa et al. 2009). However, more importantly, Bestmann et al. (2008) also showed that this contralateral decrease after TMS over left PMd during rest then becomes a contralateral increase in activation during a left-handed grip task, with stronger functional coupling following TMS (when comparing high vs. low intensity). Thus, the direction of remote effects (activation increases/decreases) was reversed depending on the state of the system. This reversal is likely caused by differences in the initial brain states, in relation to interregional mutual inhibition/facilitation mechanisms. Another demonstration of the task dependency of TMS-induced activation changes comes from O’Shea and colleagues (2007). These authors applied 15 min of offline 1 Hz rTMS over left PMd and reported compensatory activation increases in the contralateral (right) PMd. However, this effect was specific to an action selection motor task that otherwise significantly engaged (the now disrupted) left PMd. The compensation effect was not observed during a simpler motor execution task (repetitive finger movements). Importantly, when dpTMS was applied to the right PMd after 1Hz rTMS over left PMd, behavioral performance on the action selection task suffered. In other words, the compensatory right PMd activation increases after left PMd disruption were causally relevant for the task.
In a more cognitive application, Sack and colleagues (2007) revealed that TMS over the right intraparietal sulcus (IPS) only results in right hemispheric frontoparietal network effects of TMS (i.e., neural effects in local and remote regions within a functionally connected frontoparietal network) when the participant is engaged in a task that requires the proper functioning of the targeted brain region. Conversely, the authors showed that this same parietal TMS protocol did not lead to such frontoparietal network effects when the task did not rely on parietal cortex (Sack et al. 2007). Thus, parietal TMS led to significantly different local and remote brain effects depending whether, or not, the stimulated region was engaged in task-relevant processes at the time of the experimentally induced brain perturbation. These findings indicate that TMS-induced neural activity is particularly likely to spread to nodes of a (currently active) functional network and that activity does not necessarily spread to regions that are only anatomically connected to the target site. These state- and task-dependent modulations of TMS effects should not be underestimated and could also partially explain differences in remote effects between target sites when the same TMS protocol is used.
Local and Remote TMS Effects Are Functionally Relevant
The demonstration of remote neural effects of TMS raises the question of whether (and to what extent) these indirect remote effects are also relevant and functionally related to the TMS-induced behavioral changes, in other words, whether reported behavioral effects of TMS that are seemingly specific to a particular target site do actually relate to TMS-induced neural activity changes at that target site or whether these behavioral effects might relate to a widely distributed network effect. Ruff and colleagues (2006, 2008, 2009) applied TMS over right FEF inside the MR scanner and revealed remote BOLD effects in two bilateral sets of occipital brain regions within retinotopic visual areas V1–V4. Right FEF-TMS led to BOLD increases for peripheral visual field representations, but BOLD decreases for the central visual field. Assuming that higher BOLD signal equals higher-contrast sensitivity, the authors concluded that FEF-TMS may enhance peripheral, relative to central, vision. Interestingly, these behavioral predictions following the remote neural effects of FEF-TMS within early visual cortex were later confirmed by the authors in a psychophysical study outside the MR scanner. Sack and colleagues (2007) showed that simultaneous TMS/fMRI during active task execution potentially allows in vivo imaging of the neural network effects underlying TMS-induced behavioral changes. The authors applied TMS over right and left parietal cortex during whole-brain BOLD fMRI of spatial cognition performances. The authors found that right, but not left, parietal TMS (i) behaviorally impairs spatial cognition, (ii) induces neural activity changes across a right hemispheric network of frontoparietal regions, and (iii) results in significant correlations between TMS-induced behavioral impairments and neural activity changes in the directly stimulated parietal region as well as ipsilateral frontal brain regions. Thus, it appears that neural activity, not just in the stimulated right superior parietal lobule (SPL), but also in the remote ipsilateral middle frontal gyrus (MFG), was influenced by right parietal TMS (during a spatial cognition task) and contributed to a reduction in task performance (Sack et al. 2007). Importantly, these task-specific TMS-induced BOLD reductions correlated with behavioral impairment: the stronger the reduction, the slower participants responded. Again, this raises the question of whether these remote effects of right parietal TMS (e.g., at right MFG) are functionally relevant or causally related to the observed behavioral deficit. The TMS/fMRI study by Sack and colleagues (2007) does strongly suggest that the right parietal TMS-induced behavioral deficits are not exclusively caused by neural activity changes at the site of stimulation, but rather caused by neural network effects within a right hemispheric frontoparietal network consisting of right MFG and SPL. However, in this study, the functional relevance of these remote regions has to be assumed based on a correlation between the remote activation change in MFG and the behavioral impairments in spatial task performance. Therefore, in a follow-up study, the authors directly tested the functional relevance of MFG by now targeting this region directly with TMS. Causal evidence was thus provided for the functional relevance of the remote TMS activation change identified earlier (de Graaf et al. 2009). Only such an iterative approach can directly verify the functional role of revealed response profiles in distant network nodes (Fig. 8.3).
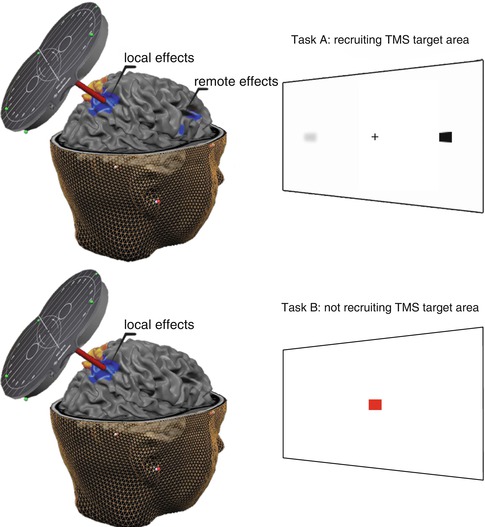
Get Clinical Tree app for offline access
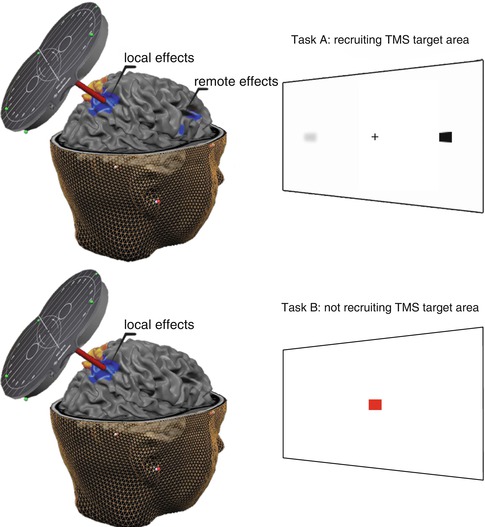
Fig. 8.3
Simultaneous fMRI and TMS during active behavior. This figure conceptualizes and generalizes the main findings of simultaneous TMS and fMRI during behaviorally controlled task execution. During execution of Task A (upper panel
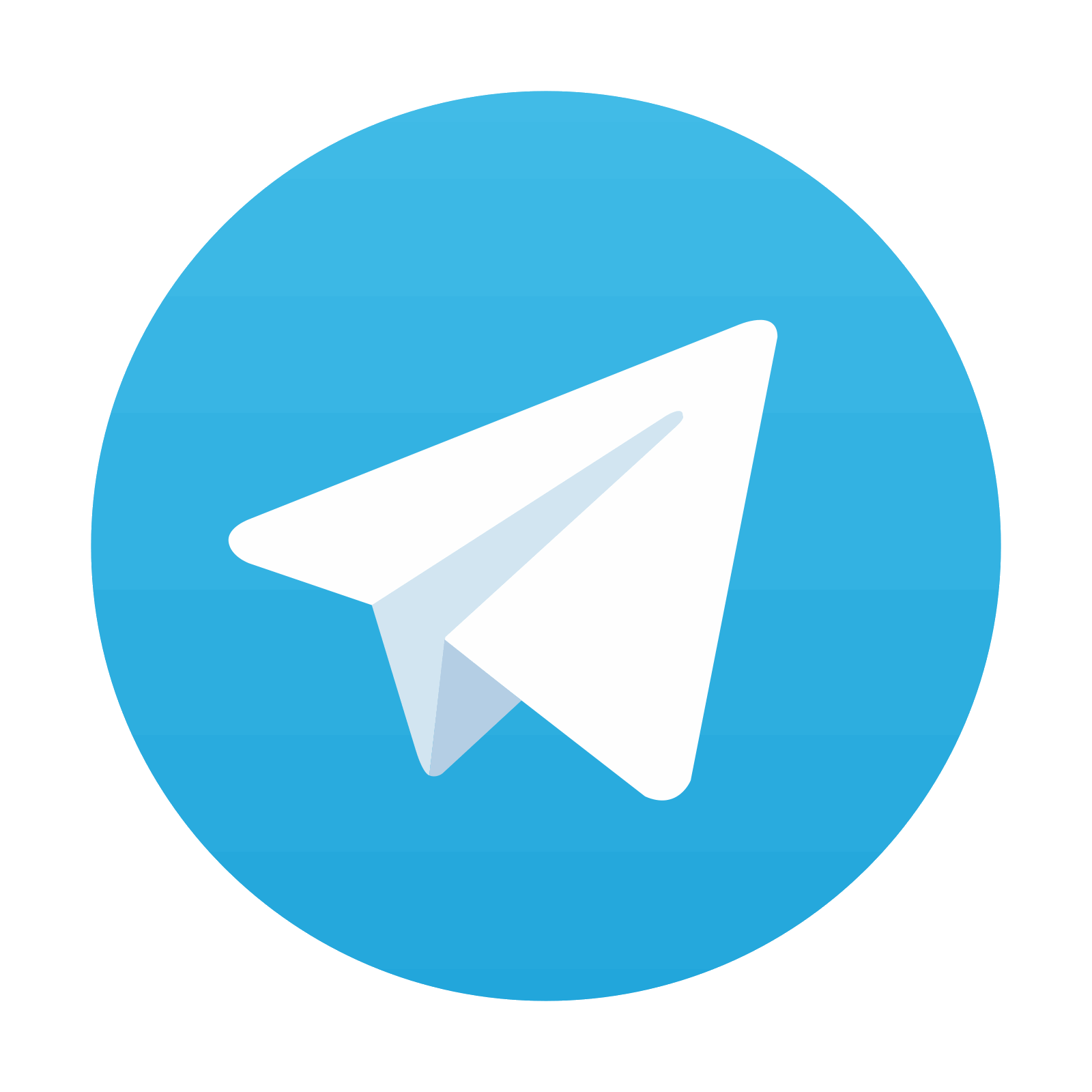
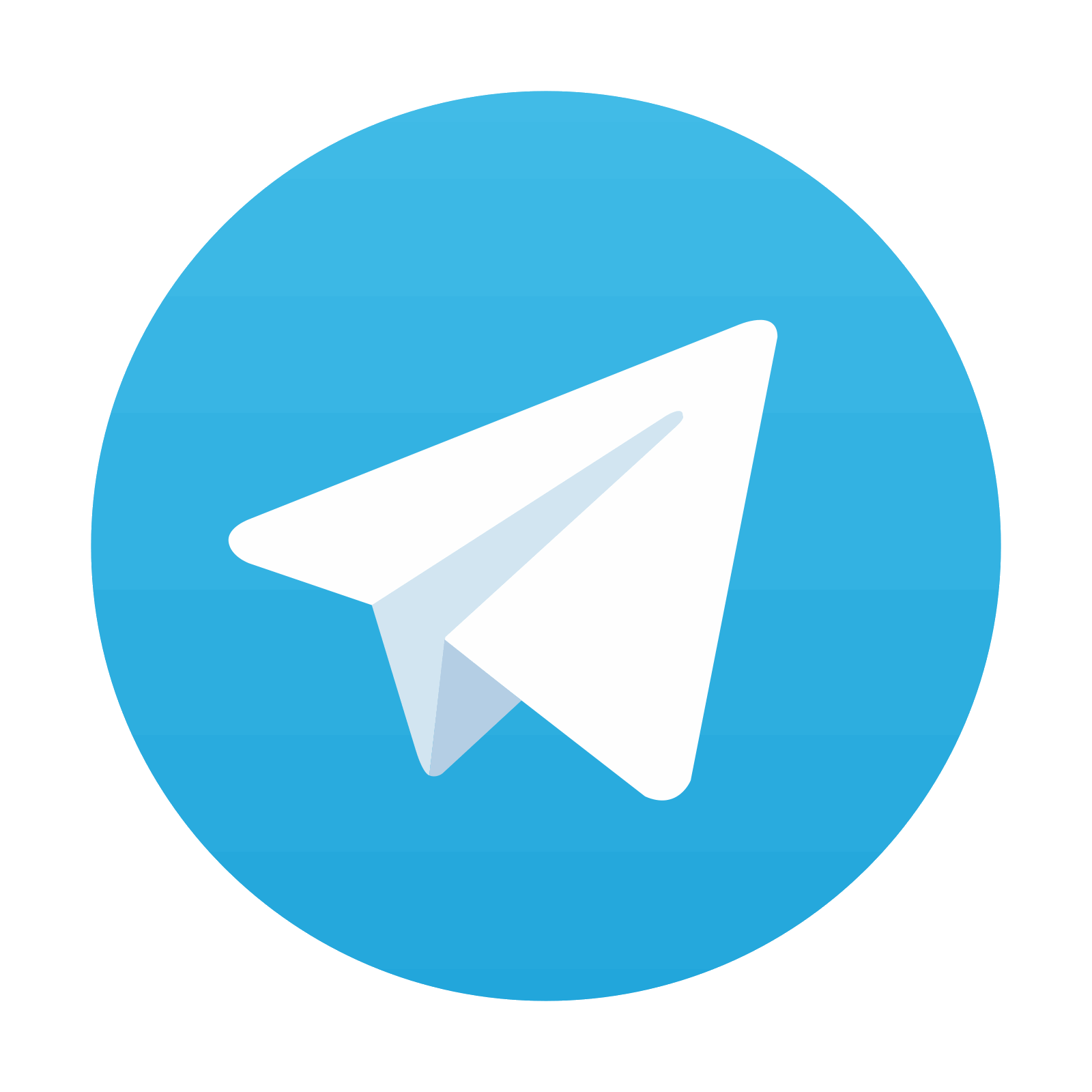
Stay updated, free articles. Join our Telegram channel
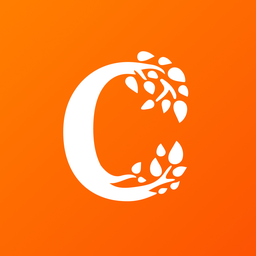
Full access? Get Clinical Tree
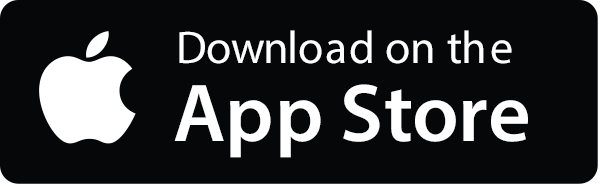
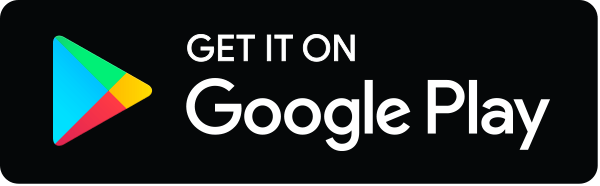
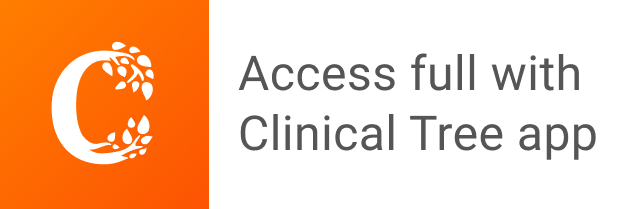