(1)
In this, and the following equations, it is important to note that we are dealing with three-dimensional vectors that describe the resulting magnetic force, as well as the magnetic field and gradient; so for example
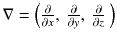



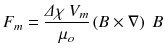
(2)

The relative magnetic susceptibility of the particle: This can be understood as a ratio of the induced magnetisation to the strength of the inducing field, and is characteristic for each type of magnetic particle in a given arrangement. During targeting experiments, the amount of force that can be exerted on the particle is proportional to Δχ, which is the difference between the magnetic susceptibility of the particle and that of the external medium. As the susceptibility of normal biological tissue and fluids, such as the blood, is usually negligible in comparison to the particle, Δχ is typically equivalent to the magnetic susceptibility χ of the particle.


The volume of the magnetic particle: (Note that we use “particle” to denote the magnetic entity that is being actuated, and that this may be a multicomponent entity, e.g. one in which many inorganic magnetic cores may be encapsulated in an organic matrix.) In cases where a cell or magnetoliposome is loaded with multiple magnetic particles and an aggregation of magnetic particles exists, the particles will typically behave as a single magnetic particle. For simplification the volume of the particles in this situation can be summed to estimate the force acting upon them:


The external magnetic field—both its strength,
, and its spatial variation (commonly referred to as the “magnetic field gradient ”), as expressed by the vector product with the gradient operator: The amount of force that can be applied to a magnetic particle is proportional to the field strength, up to field strengths that approach the saturation magnetisation (Fig. 1a) for a given particle (typically 0.5 < B < 1.0 T). Field strength depends on the strength of the magnetic device and the position of the particle relative to the magnetic field created by the device. The magnetic field gradient depends on the shape of the magnetic field that is produced by the magnetic targeting device, and the position of the particle within the field. The direction of the gradient is the main controllable parameter that will determine the direction of particle movement. In a uniform magnetic field lacking a gradient, the directional magnetic force acting upon a particle will be negligible.
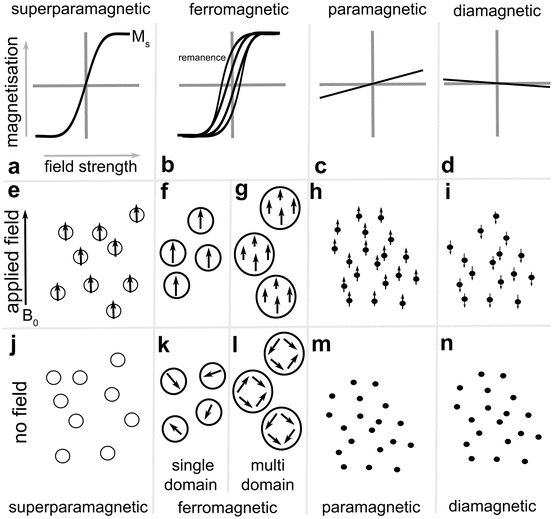

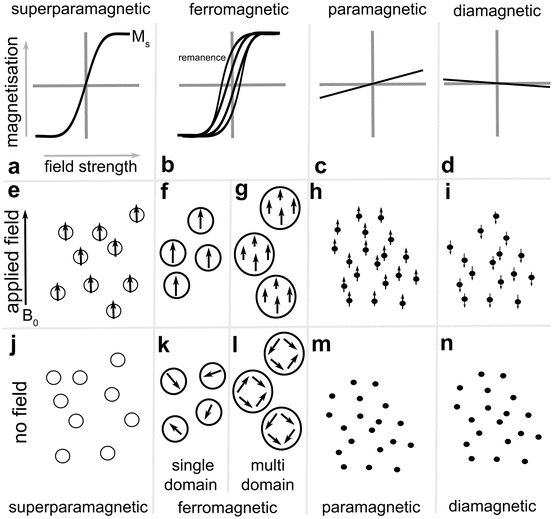
Fig. 1
Hysteresis curves showing net particle magnetisation induced by varying external field strength, for (a) superparamagnetic, (b) ferromagnetic (or ferrimagnetic), (c) paramagnetic, and (d) diamagnetic materials. Particle magnetisation in an applied field for (e) superparamagnetic, (f) single-domain ferromagnetic (or ferrimagnetic), (g) multi-domain ferromagnetic (or ferrimagnetic), (h) paramagnetic, and (i) diamagnetic materials. Net magnetisation in the absence of a magnetic field for (j) superparamagnetic, (k) single-domain ferromagnetic, (l) multi-domain ferromagnetic, (m) paramagnetic, and (n) diamagnetic materials. Further explanations on the different forms of magnetism can be found in Sect. 3.1
As the magnetic force acting on a particle is only linearly dependent on magnetic field strength over a certain range, Eq. (2) is not applicable for higher magnetic field environments, where the particle approaches its saturation magnetisation, M s. This can be visualised on the hysteresis curve (Fig. 1), which shows the induced magnetism of a magnetic particle on the y-axis in response to increasing external magnetic field strength along the x-axis. At higher field strengths, another approximation can be used to estimate the force [11]:
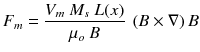
where
is the Langevin function for
, k B is Boltzmann’s constant , and T is temperature. By incorporating the Langevin function into this equation, it becomes possible to model the sigmoidal induction of magnetisation in response to increasing field strength seen on the hysteresis curve (Fig. 1), overcoming the assumption of linearity in Eq. (2). This allows Eq. (3) to be used for any field strength. Please see Riegler et al. for further details [11].
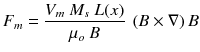
(3)
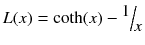
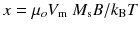
As the saturation magnetisation becomes relevant at higher field strength, this might influence the choice of magnetic material used. Of the two most common types of iron oxide used in magnetic particles, magnetite (Fe3O4) typically has higher saturation magnetism than maghemite (γ-Fe2O3), though higher values are found in some metals and alloys (Table 1).
Table 1
Bulk saturation magnetisation for selected minerals and metals/alloys at room temperature [12]
Composition | Magnetic order | M s (emu/g) |
---|---|---|
Fe3O4 | Ferrimagnetic | 90–92 |
γ-Fe2O3 | Ferrimagnetic | 70–80 |
α-Fe2O3 | Canted-antiferromagnetic | 0.4 |
CoFe2O4 | Ferrimagnetic | 80 |
MnFe2O4 | Ferrimagnetic | 77 |
NiFe2O4 | Ferrimagnetic | 51 |
Co | Ferromagnetic | 161 |
CoFe | Ferromagnetic | 235 |
Fe | Ferromagnetic | 218 |
Ni | Ferromagnetic | 55 |
Ni3Fe | Ferromagnetic | 120 |
Counteracting the magnetic force, targeting efficiency will be affected by additional physical parameters specific to the precise anatomical context, including fluid velocity (v w) and viscosity (η), difference in particle or cell velocity and fluid velocity (Δv), and particle or cell radius (R m). These can be used to calculate the drag force acting on the particle and against the magnetic force that is exerted on a particle within a given fluid [1]:
As drag force is proportional to the surface area of a particle, smaller particles will experience greater drag per unit volume than larger particles or particle-loaded cells or liposomes . Therefore the resulting force will be proportionally greater the larger the object to be targeted, assuming that it is well loaded with magnetic particles. Though in general this means that larger magnetic particles and particle-loaded entities will be targeted more effectively in the vasculature, in some circumstances this might be offset to a degree by certain advantages of smaller particles, such as increased extravasation and diffusion rate through certain tissues, which is discussed in part 3.4 of this chapter. Mathematical modelling of the forces affecting magnetic particle targeting in biological tissues has been further explored by Nacev et al. [12].
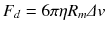
3 Magnetic Particles
3.1 Particle Size and Magnetism
Core size and material affect the type and potential strength of magnetism that a particle displays (Fig. 2), which in turn influences how it behaves in magnetic targeting applications. (Temperature also has a significant effect, but in this chapter it should be taken as read that the ambient temperature is in the room/body temperature range.) In the low nanometre range (up to 2–30 nm depending on the material [13]) most iron-, nickel-, and cobalt-based magnetic materials are superparamagnetic—they are magnetic only in the presence of an external magnetic field, and their magnetism increases with external magnetic field strength up to their saturation magnetisation (Fig. 1 a, e, j).
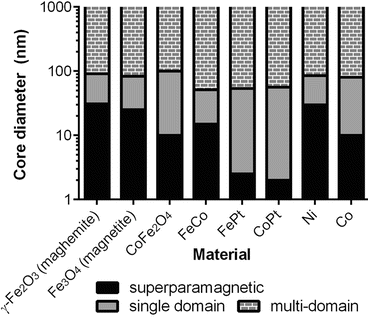
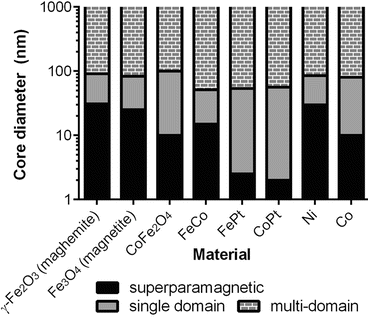
Fig. 2
Particle core size and material determine whether particles display superparamagnetism, single-domain or multi-domain ferromagnetism/ferrimagnetism [13]
Up to a higher size limit (50–100 nm, again dependent on the material [13]) the same materials will possess a single magnetic domain (Fig. 1 b, f, k). When a particle is in the single-domain state, its internal magnetisation is aligned in the same direction, whereas in multi-domain particles each domain may point in a different direction. In the application of a magnetic field, individual single-domain magnetic particles do not increase their magnetisation, which remains at saturation (M s); however, multiple neighbouring particles that are initially magnetised in varying directions (along their easy axis of magnetisation) will align with the magnetic field in chains (if in solution), or against their easy axis if they are not free to rotate. This increases the net magnetisation of the group and therefore the amount of directional magnetic force that can be applied to the particles by a field gradient .
At larger sizes, the magnetic cores will split into multiple non-aligned domains to reduce the internal energy of the particle (Fig. 1g, l). These are known as multi-domain magnetic particles. Domain wall motion can be induced by an external field to increase the net magnetisation, with domains that are magnetised in directions favoured by the applied field growing at the expense of unfavourably oriented domains, and saturation magnetisation may be reached if a sufficiently strong external field is applied. The field strength at which this is achieved, and the retention of magnetic domain alignment after removal of an external magnetic field (known as remanence ), can be determined from a hysteresis curve for the particles (Fig. 1). Though the same net effect is seen in groups of single-domain particles due to magnetic anisotropy , this is due to different underlying reasons as illustrated in Fig. 1.
The magnetic susceptibility of a given assembly of particles is influenced by the above factors, such as the composition and magnetic structure of the particle core. Magnetic susceptibility is important for targeting applications, as it is proportional to the amount of magnetic force that can be applied below field strengths at which the magnetisation is saturated. This can also be visualised on a hysteresis curve (Fig. 1). This allows us to compare magnetic properties for several potential candidate magnetic particles to determine which will be most suitable for a specific magnetic targeting application. This is particularly important when considering nanoparticles originally made for use as MRI contrast agents (superparamagnetic iron oxide particles—SPIO s), which were not designed for the effective production of force, but for their ability to produce local magnetic field inhomogeneities resulting in areas of hypo-intensity in T2-weighted MR images (due to de-phasing of the surrounding 1H proton spins). For this reason, some MRI contrast agents are not well suited for certain magnetic targeting applications due to their small core size, especially the ultra-small SPIOs or USPIOs.
Despite this potential mismatch in required design features between the two applications, some SPIOs designed as MRI contrast agents do have suitable properties for particular magnetic targeting applications. Among these are some of the SPIO particles approved for human use by the FDA, Endorem (Feridex), and Ferucarbotran (Resovist), which have both been used successfully for a number of preclinical magnetic targeting studies [2] (Table 2). Furthermore, most commercially available SPIOs have been designed for low toxicity and good cell uptake and stability, which also suits them to both cell tracking with MRI and magnetic targeting applications that use cells .
Table 2
Selection of particles used for magnetic targeting of cells
Particle name | Hydrodynamic diameter | Organs targeted | Cell types used | Core type | Reference(s) |
---|---|---|---|---|---|
Feridex/Endorem | 80–150 nm | Brain, knee joint cartilage, liver, spinal cord, vasculature | hNSC, MSC, BMSC, EPC, MNC | Multiple (5–6 nm) superparamagnetic cores | |
Resovist/Ferucarbotran | 62 nm | Femur, heart, skeletal muscle | MSC | Multiple 3–5 nm superparamagnetic cores | |
Feraheme/ferumoxytol | 17–31 nm | Heart | CDC | 6–8 nm Superparamagnetic core | [24] |
FluidMag | 50, 100, and 200 nm | Retina, vasculature | MSC | Multiple superparamagnetic cores | |
Biomag/superparamagnetic microspheres | 0.5–2 μm | Arteries, heart, vasculature | EC, CDC, MNC, | Multi-domain permanent magnetic core (0.5–2 μm) | |
Magnetospirillum sp.-derived particles | ~40 nm | Hind limb | EPC | Single-domain permanent magnetic core (~40 nm) | [28] |
Multifunctional upconversion nanoparticles | 240 nm | Skin wound | MSC | Multiple 5 nm superparamagnetic cores | [29] |
3.2 Particle Aggregation
Particle aggregation can be promoted by both biological and magnetic factors, and might be beneficial or detrimental according to the type of magnetic targeting application and the duration and scale of aggregation. As superparamagnetic particles are magnetic only in the presence of a magnetic field, they will not magnetically aggregate in zero field. However, temporary chains of superparamagnetic particles can form at sufficiently high concentration and field strength as a result of attractive dipole-dipole interactions [14]. Permanent magnetic particles however will cluster together in the absence of an external magnetic field, and will form chains parallel to an applied external field. Clustering and the formation of chains in particles and particle-loaded cells are also dependent on their concentration, surface charge and chemistry, and viscosity and flow rate of the solution. This can be modelled for a given scenario to predict the optimal parameters to use [30, 31].
For some applications requiring the production of vascular embolisms, particle aggregation is desirable, and larger magnetic particles into the micron range or above have been used for this purpose [32, 33]. For other applications such as drug or cell delivery that require even distribution throughout a tissue and efficient extravasation, aggregation will impede delivery and so the use of smaller single-domain or superparamagnetic particles might be more effective [34]. However, the tendency of particles to form temporary chains or small aggregations during delivery will increase the amount of force that can be applied by an external field and can increase targeting efficiency, so this must be balanced against the probability of embolization to achieve optimum delivery. In addition to tuning particle size, the balance between the application of sufficient force and the possibility of embolization can be achieved by assessing a range of targeting device field strengths for a given application [22].
Recent work has also suggested that microfabricated particles comprising multilayer stacks of alternating magnetic and non-magnetic materials can be used to avoid aggregation. These can be produced with diameters on the scale of multidomain ferromagnetic particles (2 μm), but with zero remanence, and with an efficient switch to saturation magnetisation upon application of a given field strength [35]. This avoids some of the limitations associated with the aggregation of larger particles while retaining many of the benefits including the higher amount of force that can be applied per particle and increased circulation times (Sect. 3.4). The construction of larger composite particles from multiple small superparamagnetic cores can also avoid the magnetic aggregation typical of larger permanently magnetic particles while retaining the benefit of higher magnetic force per surface area and thus increased overall targeting efficiency. Several other methods can achieve similar effects , including the creation of “nanoworms” from strings of particles [36, 37], or embedding multiple superparamagnetic cores within dextran [38] or silica coating [39], hydrogel, [40], vesicles [41], or magnetoliposomes [42].
3.3 Cell Uptake and Labelling
Particle size also affects cell uptake, and therefore the resulting amount of force that can be applied to a particle-loaded cell will be determined not only by the particle core size, but also by how many particles the cell has taken up. Around ten different biological mechanisms of particle uptake have been discovered in cells, and are active to varying degrees depending on the specific cell type and its environment, and the size, charge, and coating of the particle [43, 44]. These include non-specific uptake mechanisms such as macropinocytosis (particles around 1 μm) and caveolar-mediated endocytosis (for particles ~60 nm), as well as receptor-dependent mechanisms such as clathrin-mediated endocytosis (~120 nm) and clathrin-and caveolin-independent endocytosis (~90 nm) [45]. Due to the complexity of cell uptake mechanisms, it is difficult therefore to predict with accuracy the range of particle sizes that will be taken up effectively by a given cell type, except for cells that have already been investigated with similar particles. Generally, particles between 20 and 200 nm in diameter are more effectively taken up by most non-phagocytic cell types than particles either side of that range [46–48], though increased particle charge will improve the uptake of larger particles into the micron scale [48]. In addition to this, other interacting variables such as particle shape, stiffness, and surface chemistry will affect the uptake. A complete discussion of this topic falls outside the scope of this chapter and the reader is referred to a recent comprehensive review [44].
As most commercially available and FDA-approved magnetic particles designed for imaging applications fall within this range of 20–200 nm, and have been optimised for cell uptake by size and surface coating, these have found successful use in several magnetic cell-targeting studies [2] and can be used for reference in designing particles for this purpose (Table 2).
When labelling adherent cells with magnetic particles, uptake can sometimes be promoted by application of a magnetic field. This has been demonstrated with a range of particle and cell types [49, 50]. Additionally, this technique can be used to simultaneously introduce DNA to the cell (known as magnetofection), which might be useful if the cells are to be used for gene therapy [51].
Addition of antibodies to the particle surface can also promote uptake or attachment to specific cell types, according to the choice of antibody. In this way, cells can be labelled either prior to implantation or in vivo. For example, antibody-labelled magnetic particles administered intravenously can label specific cell types within the circulation, allowing these cells to be magnetically targeted to specific locations within the body for therapeutic purposes. This strategy has been demonstrated to improve repair of infarct myocardium using circulating stem cells [6]. Here a dual mechanism of targeting was demonstrated, in which cells were targeted to the heart using a magnetic field, and also by the particles that displayed antibodies for both the stem cell and for the damaged cardiac tissue. As this approach required only the intravenous administration of the particles, translation would be more straightforward than in therapies in which cells must be harvested, isolated, and labelled before being given to the patient. This strategy might also prove effective for magnetic targeting of antibody-based drugs, or together with the delivery of other small-molecule drugs or therapeutics such as siRNA (see Chap. 7) contained in magnetoliposomes or adsorbed to the particle surface.
3.4 Targeting Particles for Drug and Therapeutic Delivery
The required design features of particles that are to be used directly for therapy differ from those that are to be used to label cells, as do the constraints on these. This is due to several biological and physical factors.
For example, particle size affects extravasation rate and diffusion though tissue, and will affect the ability to target regions distant to the vasculature. This may be a particular concern for certain applications such as drug delivery to poorly vascularised metastases, or the targeting of particles for hyperthermia therapy. The effect of particle size on diffusion and extravasation can be modelled in a number of ways, and used to predict the range of particle sizes that might benefit from magnetic targeting, or which would be effective in a given tissue. In cases where particle size falls outside this range (20–400 nm, based on a simulation of tumour tissue), delivery by endogenous diffusion mechanisms might prove superior to targeting strategies [34].
Clearance of particles by the reticuloendothelial system (RES) and renal filtration are also dependent on size. Renal filtration will quickly clear the majority of circulating particles under 5 nm, but barely any that are above 9 nm [52]. Larger particles can be cleared by immune cells including Kupffer cells in the liver, monocytes, spleen, and macrophages, which engulf nanometre- to micron-sized particles, decreasing delivery to the target organ. Targeting efficiency will therefore be improved the faster the particles can be attracted to the target site, before clearance has occurred, though this may be challenging when most particles are cleared on the first-pass circulation post-injection. The rate at which particles are scavenged in this way is affected by their coating and size. It is outside the scope of this chapter to review this topic in detail, but several modifications can reduce RES uptake such as polyethylene glycan (PEG) coating , which has been demonstrated to enhance the circulation time of particles and improve magnetic targeting efficiency [53]. The utility of PEGylation in reducing RES uptake is further illustrated by the results achieved with Doxil—a liposomal formulation of doxorubicin , and the first nano-encapsulated drug to obtain clinical approval [54].
Another strategy in reducing RES uptake utilises the properties of red blood cells, which naturally evade clearance and achieve long circulation times through a combination of their size, surface composition, and charge. For this reason they have been used in magnetic targeting applications for drug delivery [55, 56]. In one study red blood cells were loaded with a drug for photodynamic therapy and targeted to the tumour, resulting in a decrease in tumour volume following phototherapy compared to non-magnetically targeted control conditions [56]. In an adaptation of this concept, nanoparticles can be coated with extracted cell membrane of red blood cells, thereby “cloaking” the underlying material. This provides a strategy to utilise the unique binding properties of red blood cells to diseased tissue and pathogens [57], and could facilitate particle-based drug delivery to sites of inflammation in combination with magnetic targeting.
On the other hand, several iron oxide contrast agents for MRI, including those that have been clinically approved, were originally designed with size and surface coatings to optimise their uptake by the RES, for the purpose of imaging uptake in the liver. These might not therefore be optimal for magnet-targeted delivery of drugs due to their increased clearance rate .
To bypass normal clearance mechanisms, several magnetic targeting studies have opted for local delivery to the target organ using a directed injection [4, 26, 58], instead of a systemic injection. For example in a clinical trial targeting hepatocellular carcinoma, particles were injected via a catheter inserted into the hepatic artery, which could be moved intraoperatively between the acquisition of magnetic resonance images to ensure that particles were effectively delivered to the entire tumour (Fig. 3) [3]. This strategy can reduce the number of particles or cells that can be cleared by the RES prior to reaching the site of interest, increasing the efficiency of delivery. Though this approach increases the invasiveness of the technique, it may be required in several situations to achieve effective delivery. However, some difficult-to-target organs such as the brain have been efficiently targeted using a systemic intravenous injection of particles [38], though this may depend on blood-brain-barrier breakdown related to specific disease states. Together, these results suggest that both systemic and local delivery can be effective.
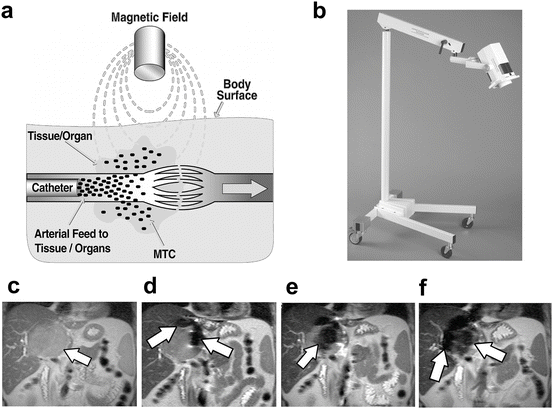
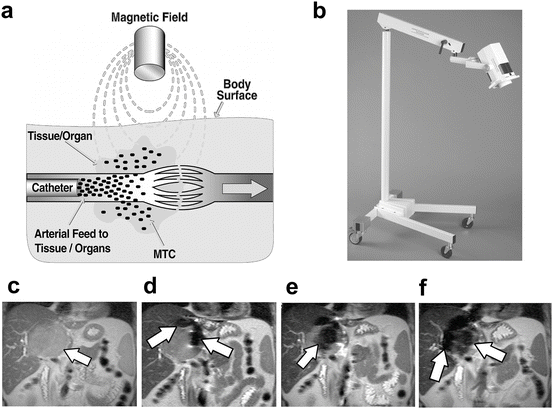
Fig. 3
(a) Diagram depicts the mode of action of magnetic targeted therapy. After leaving the intra-arterial catheter, magnetically targeted carrier (MTC) doxorubicin is drawn out of the artery into surrounding tumour and/or liver tissue by the influence of the local magnetic field (image courtesy of FeRx). (b) 5 kG portable magnet used for magnetic targeted therapy. The overall height of the magnet and holding apparatus is 1.4 m. (c–f) Coronal single-shot spin-echo MR images (839/80, one signal acquired, 6 mm section thickness) obtained, c, before MTC-DOX administration and, d, after the first, e, the second, and, f, the third dose of MTC-DOX. The selective hepatic arterial catheter was repositioned between each dose [3]. Figure reproduced from Wilson et al. Hepatocellular carcinoma: Regional therapy with a magnetic targeted carrier bound to Doxorubicin in a dual MR imaging/conventional angiography suite—Initial experience with four patients. Radiology 2004;230:287–293, with permission from the Radiological Society of North America
Particle size also affects the surface area-to-volume ratio, which is relevant to the adsorption of drugs to the particle surface. However, there is a trade-off between the motivations of using smaller particles, and the increased force that can be gained from using larger particles, and so a range of particle sizes can be considered. For example, magnet-targeted drug delivery in the clinic has been trialled across a scale of particle sizes: 100 nm particles coated with glucose and bound to epirubicin [4], and 0.5–5 μm sized particles with an activated carbon coating bound to doxorubicin [3]. Even larger metallic objects in the millimetre scale have been used for targeting and hyperthermia therapy, which does not require the same extent of extravasation and distribution of particles, due to transfer of heat across tissue [7].
For targeting tumours, particle retention can be improved by the enhanced permeability and retention (EPR) effect , which has been well documented to improve delivery [59]. As this effect relies on leaky vasculature and diffusion of particles through the extracellular space, it is a size-specific phenomenon, with the enhancement of particle retention decreasing with particle diameters above 30 nm in one tumour model [60], though this will differ to some degree depending on tumour type and nature of its vascularisation. Though enhanced nanoparticle delivery is achievable in difficult-to-access sites, such as brain tumours with magnetic targeting [61], one limitation of magnetic targeting for cancer treatment is the issue of metastases, which can be remote, disperse, and difficult to identify. These factors would limit the ability for targeting to be used, as they might require a separate targeting field to be applied for each site that could be identified .
For drug delivery it is also necessary to consider the attachment of cargo to the particle. In the simplest form this can rely on absorption of the drug onto the particle surface [3, 4]. However controlled release of the drug can be achieved if it is trapped in a solid coating, such as silica, around the magnetic particle. By heating the particle using a focused alternating field around the target tissue, as during a hyperthermia treatment, the coating can be made to melt, thereby releasing the drug [5, 41]. Similar strategies can be employed to release drugs from magnetoliposomes [62].
4 Magnetic Devices for In Vivo Targeting
Appropriate selection or design of magnetic targeting devices depends largely on the anatomical region to be targeted. Other considerations include the sort of particle that will be targeted (small superparamagnetic particles will typically require a stronger magnetic field and field gradient to exert the same force on them than larger or permanent single-magnetic-domain particles), as well as the type of steering and/or imaging that is required for the application. Several broad categories of magnetic targeting device will now be discussed, including their benefits, limitations, and applications to which they are suited.
4.1 Permanent Magnets
Small permanent magnets have found wide use in preclinical magnetic targeting studies, as they are readily available and provide high field strengths and gradients over a relatively small area, without the larger hardware size or cooling required for electromagnets. While suitable for tissue depths of a few centimetres, the limited spatial extent of such fields would restrict their external use in the clinic to targeting superficial tissue [3, 4], unless the particles are delivered to the internal site by direct injection (Fig. 3). Furthermore, a single permanent magnet cannot produce a local maximum of field gradient within the patient (unless surgically implanted—see below), meaning that for practical purposes that magnetic particles will be attracted from the inside of the patient to the surface of the magnet to produce a focal accumulation towards the skin, rather than vice versa. This makes it difficult to target an internal location non-invasively or achieve an even distribution of particles throughout a target organ. One solution to this is to change the location of the magnet during the targeting procedure , for example from one side of the liver to the other, so that the targeted particles are more evenly spread out [34]. This was demonstrated ex vivo to increase the concentration of magnetic particles within poorly perfused liver metastases by 1.8-fold above diffusion alone, and was predicted to be effective for particles in the 30–500 nm diameter range, with normal diffusion acting more effectively than targeting for smaller particles [34]. Another option is the implantation [18] or stereotactic insertion of magnets [32, 33]. While this can achieve effective targeting to internal organs, it does not completely avoid the invasive procedures associated with more traditional surgical implantation methods. Alternatively, magnetic stents can be manoeuvred to a specific point of the vasculature to achieve targeting of cells or particles [63], but again this is invasive and only useful for targeting areas that are accessible in this way.
4.2 Permanent Magnet Arrays
By superimposing two or more magnetic fields in an array of permanent magnets, some of the limitations of single permanent magnets can be overcome to produce more complex field shapes and gradients. With certain configurations this can allow the device to remain external to the target tissue and steer magnetic particles away from the magnet itself. A simple example is two bar magnets that have been arranged so that their fields overlap to create a remote point from which magnetic particles are pushed away from the magnet (Fig. 4). This has been posited as a solution for magnetically “injecting” nanomedicines into the inner ear [64], and has been validated in a rat model over distances relevant to use in the human ear [65]. This is important as the ear is behind the blood-brain barrier and can be a difficult organ in which to achieve drug delivery. It is also protected by the tympanic, round window, and oval membranes, and magnetic targeting using a “pulling” magnetic field from the opposite side of the head would require an unfeasibly strong magnetic field to achieve the same amount of force [64].
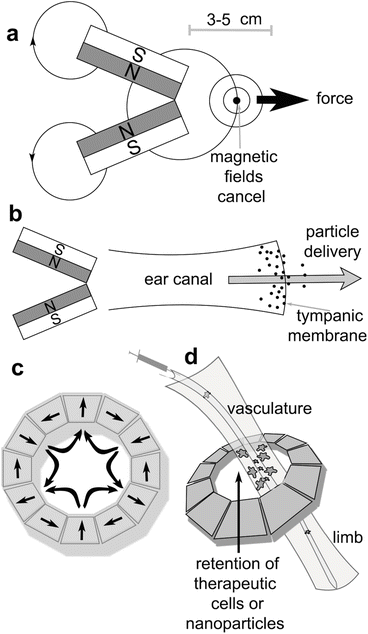
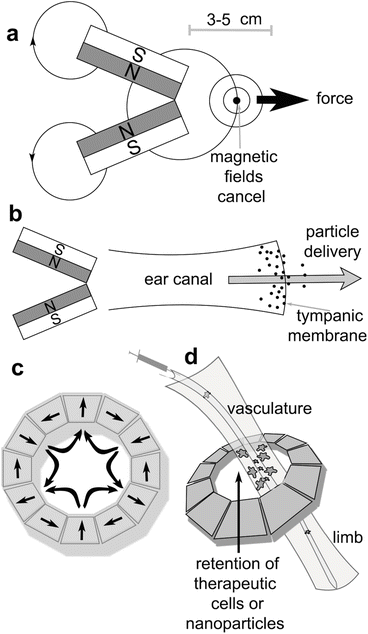
Fig. 4
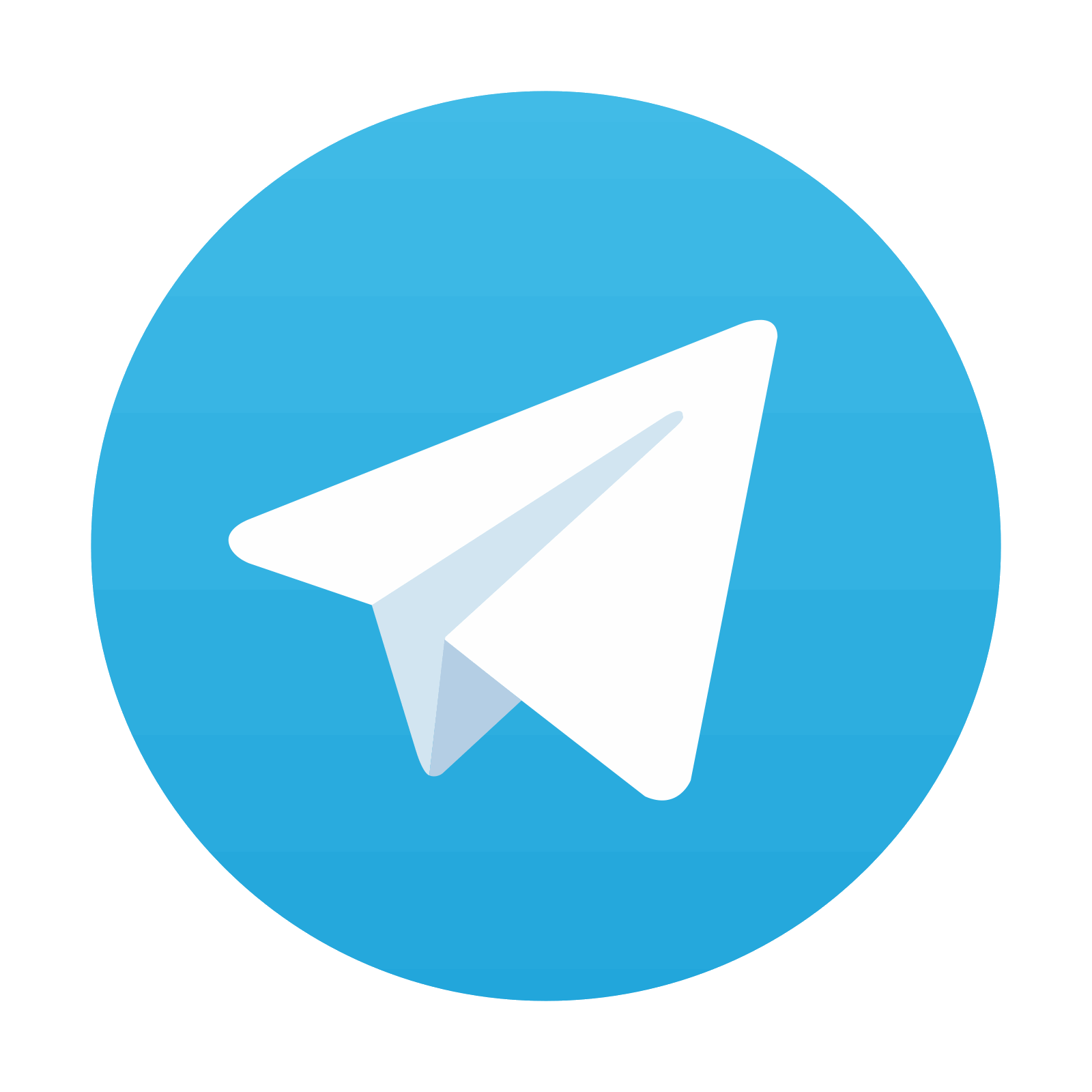
(a) Two permanent magnets arranged at an angle can create a remote focal point about which magnetic particles may be repelled. (b) This magnet configuration has been proposed as a way to deliver magnetic particles to deep-tissue locations such as the inner ear [63, 64]. (c) Cylindrical Halbach array of permanent magnets [26], where the arrows show the direction of force. (d) The Halbach array pictured in (c) can be used to retain therapeutic cells within the vasculature, providing potential regenerative effects in cases of peripheral limb ischemia [11, 26]
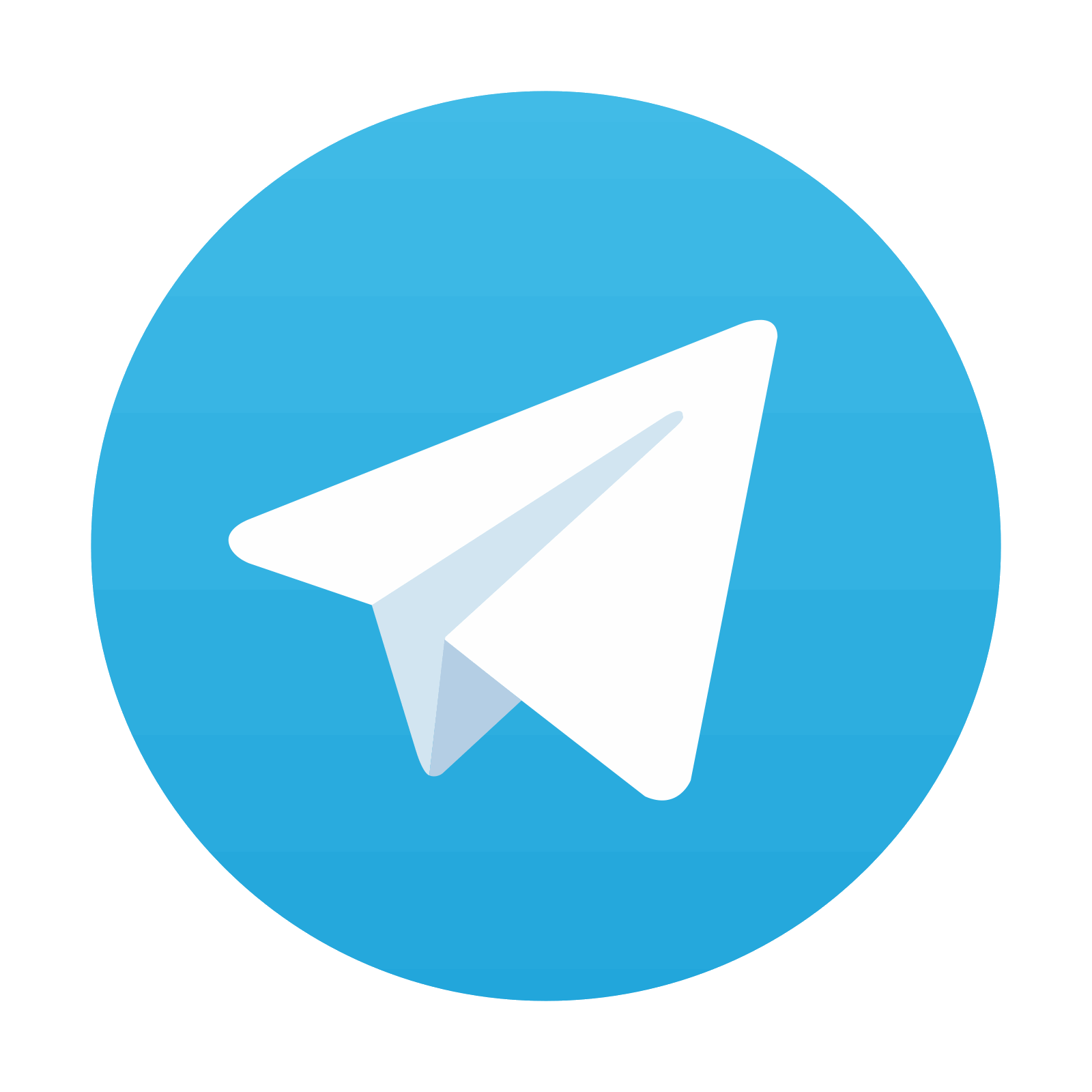
Stay updated, free articles. Join our Telegram channel
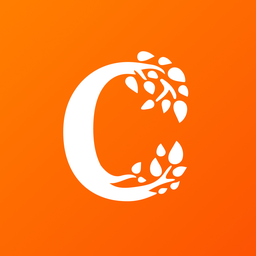
Full access? Get Clinical Tree
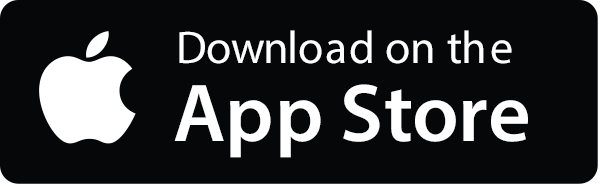
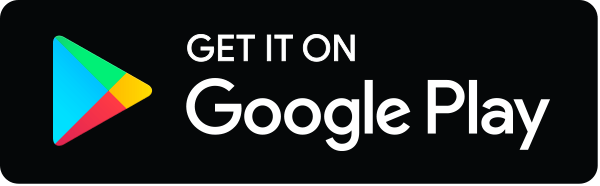
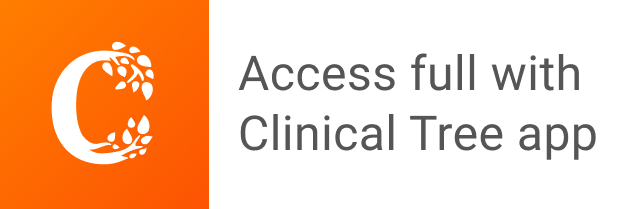