(1)
where I is the transmitted beam intensity, I 0 is the incident beam intensity, and x is the thickness of the medium. The mass attenuation coefficient, μ, expressed in cm2/g, is best described by the following formula:

(2)
Modern intravenous X-ray imaging agents, first appeared in 1954, are all based on the 1,3,5-triiodobenzene structural platform [11–13]. Because of the superior water solubility, versatile synthetic chemistry and high tolerance by the human body, such iodinated organic compounds have dominated the clinical application of X-ray imaging agents with ionic or covalent derivatives of tri-iodinated benzene compounds. The iodinated organic compounds are characterized by a nonspecific extracellular distribution and a short imaging time of approximately 1.5 h due to their rapid clearance by the kidney [13]. The rapid pharmacokinetics of iodinated organic CT imaging agents has thus limited their microvascular and targeting performance [11, 12]. From the viewpoint of contrast enhancement, use of iodine as an X-ray imaging agent is not optimal because its X-ray attenuation effect is rather low compared with the typical metal-based counterparts [2, 3]. The current use of iodinated X-ray imaging agents is mainly based on their superior safety and cost, rather than on their X-ray attenuation effects [13, 14]. In this regard, bismuth-based materials represent a unique opportunity for the development of a new generation of X-ray imaging agents.
2 Physical, Chemical, and Biological Properties of Bismuth
Bismuth (Z = 83) is a soft gray metal that occurs in nature commonly as bismite (Bi2O3), bismutite ((BiO)2CO3), or bismuthinite (Bi2S3). The metallic form of this element is usually obtained as a byproduct from copper, lead, tin, molybdenum and tungsten smelting. Bismuth is a relatively rare element with the abundance in the Earth’s crust estimated to be about 0.2 ppm, approximately about twice as abundant as gold. When freshly produced, it is a brittle metal with a silvery white color, but often exhibits a pink tint after exposure to air due to surface oxidation. Among metals, bismuth is the most diamagnetic element, has the lowest thermal conductivity, and exhibits the highest Hall effect. Because of its wide temperature range between the melting point (T melt = 271 °C) and boiling point (T boil = 1560 °C), and low neutron absorption cross section, liquid bismuth is used as a coolant in nuclear reactors. The density of bismuth, d = 9.78 g/cm3 is ~86 % of the density for lead, d = 11.32 g/cm3, making bismuth useful as a nontoxic replacement for lead in ceramic glazes, fishing sinkers, food-processing equipment, free-machining brasses for plumbing applications, lubricating greases, and ammunition for waterfowl hunting [15]. There is only one naturally occurring isotope of bismuth,
that has long been considered as the heaviest stable isotope. In 2003, however,
was found to decay via alpha-emission with a half-life to be 1.9 × 1019 years, more than a billion times longer than the current estimated age of the universe [16]. For all practical purposes, bismuth is still treated as nonradioactive.


Bismuth has two oxidation states, Bi(III) and Bi(V). The latter is a powerful oxidant in aqueous solution with a redox potential of E° = 2.03 V for Bi(V)/Bi(III). Under the physiological conditions, Bi(III) is the most common and the only stable form of the element. The inorganic Bi(III) salts of sulfate, perchlorate, nitrate, as well as halides, except fluoride, all appear to be soluble in water, but hydrolyze to form insoluble hydroxide or oxyhydroxide products in neutral solution [15]. In acidic solution, such hydrolytic reactions are complex, and products formed are sensitive to the pH value, as well as the presence of different counter ions. These products are commonly referred to as “basic,” “oxy-”, or “sub-” salts with the formation of the bismuthyl ion (BiO+) or various polynuclear clusters of low aqueous solubility [17]. As a heavy metal, the toxicity of bismuth is much lower than that of its heavy-metal neighbors, such as mercury, thallium, lead, and polonium [18]. Because of its low solubility in the blood, bismuth is readily cleared from urine, and exhibits no long-term carcinogenic, mutagenic or teratogenic effects. Its biological half-life for whole-body retention is about 5 days, although it may remain in the kidney for years in patients treated with bismuth compounds [19].
The use of bismuth compounds in medicine can be traced back to the Middle Ages, although the first full account of such use did not appear until 1786 for the treatment of dyspepsia [19]. A decade later, bismuth compounds were used as antisyphilitic drugs. In the late nineteenth century, a variety of bismuth compounds mixed with iodoform were promoted as a surgical wound dressing owing to its antimicrobial and antibacterial properties. The use of various bismuth compounds, such as subnitrate, subgallate, subcitrate, subcarbonate and subsalicylate to treat syphilis, hypertension, infections, skin conditions and gastrointestinal disorders lasted until 1950s after penicillin and several other antibiotics had become widely available [18]. Currently, the only continued medicinal application of bismuth is for the treatment of diarrhea and dyspepsia using bismuth subsalicylate (Pepto-Bismol®; the Procter & Gamble Company, Cincinnati, OH, USA), or bismuth subcitrate (De-Nol®; Gist Brocades, Delft, The Netherlands) [18, 19]. On the other hand, bismuth oxychloride (BiOCl) found in nature as the mineral bismoclite has been used as a pigment in cosmetics since the ancient Egyptian Times. Owing to its layered structure, BiOCl refracts light chromatically, resulting in an iridescent appearance resembling nacre of pearl [20].
3 Development of Bismuth-Based Nanoparticles for CT Imaging
Bismuth compounds have high X-ray attenuation efficiency, and thus can provide sufficient contrast enhancement at low dosage levels when they are employed as CT imaging agents. Furthermore, the biocompatible and nontoxic nature of bismuth represents an extremely attractive feature for many molecular compounds of bismuth to function as effective CT imaging agents. However, the very first requirement of an effective CT imaging agent is the high solubility of the compound, as a high concentration is necessary for an intravenous administration to produce sufficient contrast in CT imaging due to the intrinsic low contrast sensitivity of the X-ray CT modality. Additionally, the typical ionic, covalent or coordination complexes of bismuth may bind to proteins or other biomolecules or undergo transmetallation reactions with endogenous ions, such as Ca2+, Mg2+, Mn2+, and Zn2+, thus altering their pharmacological characteristics when administrated intravenously.
Use of bismuth-based nanoparticles, rather than bismuth-containing ionic or covalent compounds, to develop CT imaging agents have potential to circumvent the high-osmolality or poor-solubility limitations of its ionic or covalent compounds. It should be noted that thus far the small-molecule platform has not yet afforded clinically useful bismuth-containing CT contrast agents. On the other hand, there has been an important paradigm shift to use various nanoparticles for the development of new-generation imaging agents in different imaging modalities including X-ray CT imaging [21, 22]. Additionally, particulate-imaging agents can have a greater blood circulation half-life than the molecular counterparts, thus increasing the time window for imaging [23]. Furthermore, surface-modifications via ionic or covalent attachment of a targeting agent on nanoparticles provide possibilities to develop organ-specific imaging agents [24, 25].
3.1 Polymer-Coated Bi2S3 Nanoparticles for CT Imaging
Weissleder and coworkers were the first to explore the application of nanoparticles for CT imaging in small animals [26]. In 2006, they reported on the development of a polymer-coated Bi2S3 nanoparticle preparation as an injectable CT contrast agent. They developed a two-step synthetic method to prepare homogeneous preparations more suitable for parenteral use than those previously reported. Nanocrystals of bismuth sulfide were first grown by precipitation from the reaction between bismuth citrate and sodium sulfide in the presence of a thiol surfactant. The nanocrystals were then coated with polyvinylpyrrolidone (PVP) to afford PVP-coated Bi2S3 nanoparticle (BPNPs) .
The TEM images revealed that such BPNPs have a quasi-rectangular platelet shape with the length ranging from 10 nm to about 50 nm per side. The thickness of the crystals is 3–4 nm. The mean hydrodynamic diameter of BPNPs was measured to be 30 ± 10 nm by laser-light scattering (Fig. 1).
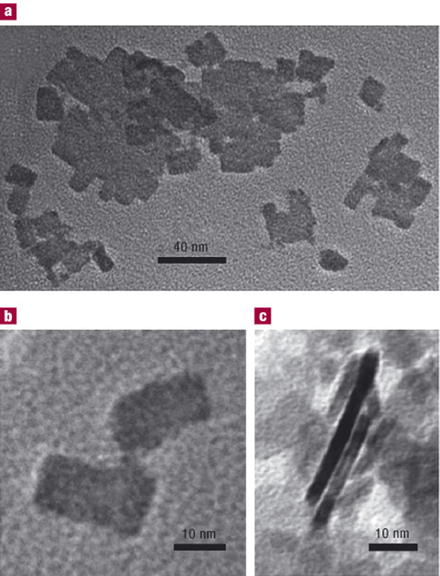
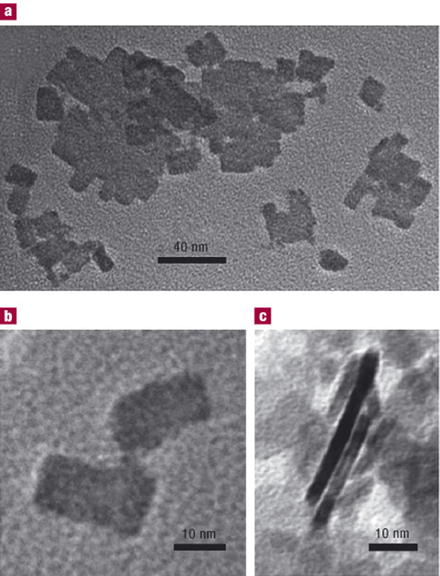
Fig. 1
BPNP characterization. TEM characterization of the Bi2S3 nanoparticles
The CT opacity measurements showed that that a BPNP preparation of 0.55 M in bismuth concentration is equivalent in X-ray absorption to a 2.36 M iodine solution. After intravenous administration of 250 μl BPNPs (~57 μmol Bi3+) into Balb/c mice, serial CT imaging was performed to determine vascular enhancement and half-life. It was found that the blood signal increases from −27 ± 77 HU to 530 ± 150 HU at its peak. The enhancement provides clear delineation of the cardiac ventricles and all major arterial and venous structures (Fig. 2).
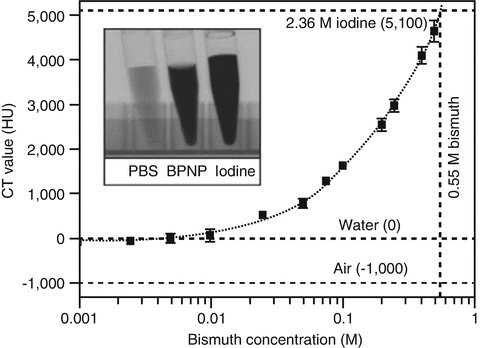
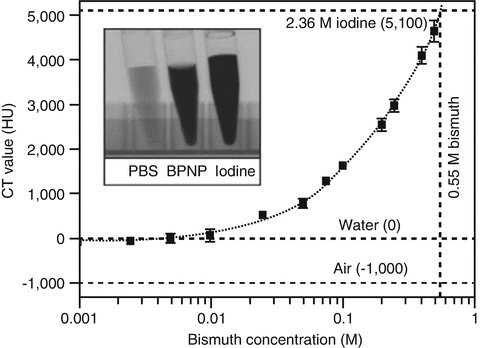
Fig. 2
X-ray absorption of BPNPs. Experimental CT opacity of bismuth solutions as a function of concentration. The horizontal dashed lines indicate the opacities of air, water and 2.36 M (300 mg × ml−1) iodine contrast agent, for comparison. Inset: X-ray fluoroscopy of tubes containing PBS, 0.5 M Bi2S3 nanoparticle suspension (BPNPs) and 2.36 M iodine contrast agent (Iopromide). The error bars represent the standard deviation in the CT value of a set of 30 × 30 × 1 (900) voxels on a planar square aligned with the center of the sample receptacle
The blood half-life of BPNPs in mice was determined to be 140 ± 15 min, which is significantly longer than for commercial iodinated preparations (<10 min). Furthermore, their extended imaging studies at 12–24 h after intravenous administration showed that the BPNPs were distributed to organs containing phagocytic cells (liver, spleen, lymph nodes). They found that liver signal intensity increased from −22 ± 77 HU to 740 ± 210 HU at 24 h (Fig. 3d), which probably reflects uptake of BPNPs into macrophages (Kupffer cells) and hepatocytes. In the meantime, organs devoid of major phagocytic cells showed no or only a slight enhancement. Weak contrast in the kidneys and urinary tract was visible 1–2 days after the administration of BPNPs, presumably due to renal excretion. In preliminary observations, they found no residual bismuth in any organ 2 months after the administration of a single dose. In order to investigate if BPNPs could be used for lymph-node delivery to improve cancer staging, mice were injected subcutaneously with 50 μl BPNPs (~11.4 μmol Bi3+) and repeatedly imaged up to 140 h after injection. Regional lymph nodes were clearly contrasted (Fig. 3).
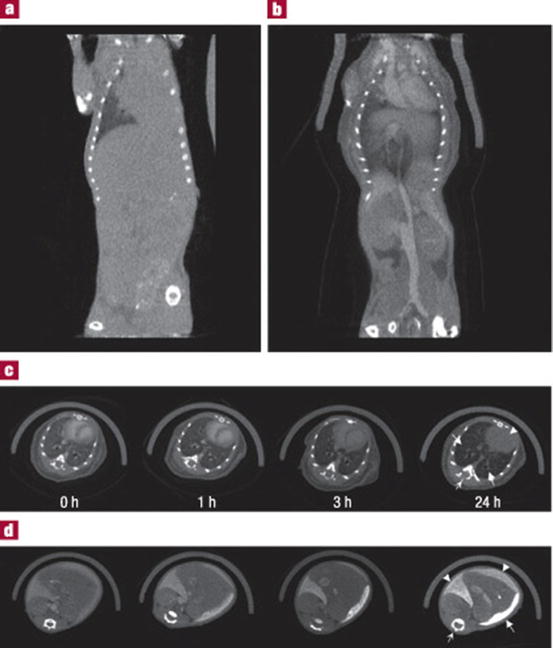
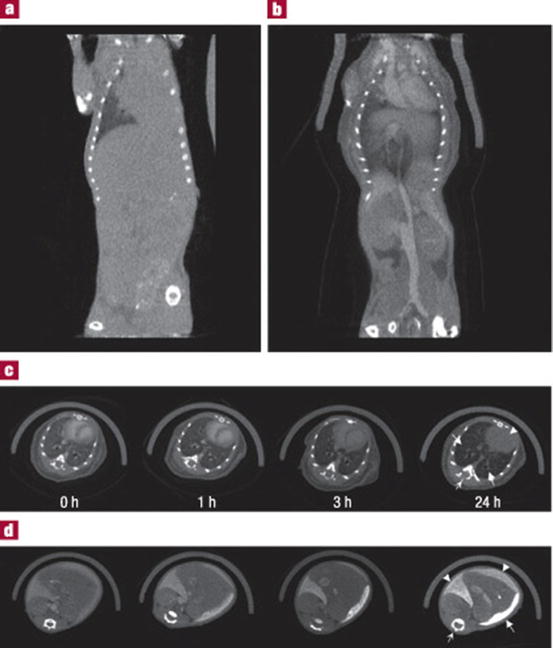
Fig. 3
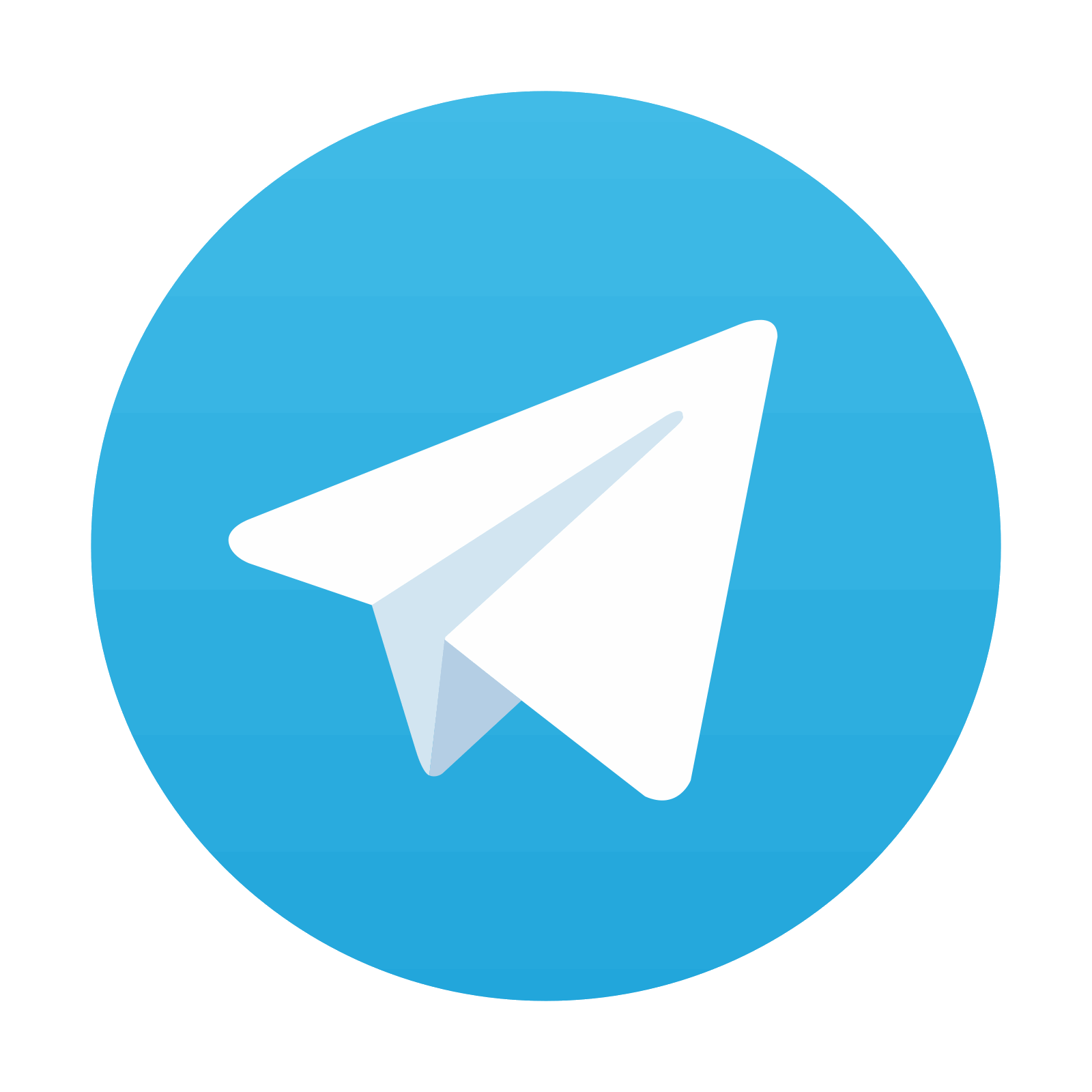
Serial in vivo imaging of vasculature. (a, b) Coronal curved multiplanar reformatted reconstructions of a live Balb/c mouse before (a) and after (b) intravenous BPNP administration, showing the vascular enhancement of large vessels and the heart, and the organ delineation, achieved with the nanoparticle agent. The length of the reconstruction in a and b is 6.5 cm. (c, d) Serial CT scans of a live Balb/c mouse following tail-vein injection of 250 μl BPNP suspension (0.228 M bismuth). Series c shows transverse slices through the heart (arrowhead), the lungs (solid arrow), and a vertebra (open arrow). Series (d) are transverse slices including the liver (arrowhead), the spleen (solid arrow), and a vertebral body (open arrow). The diameter of the mouse in c and d is 1.6 cm
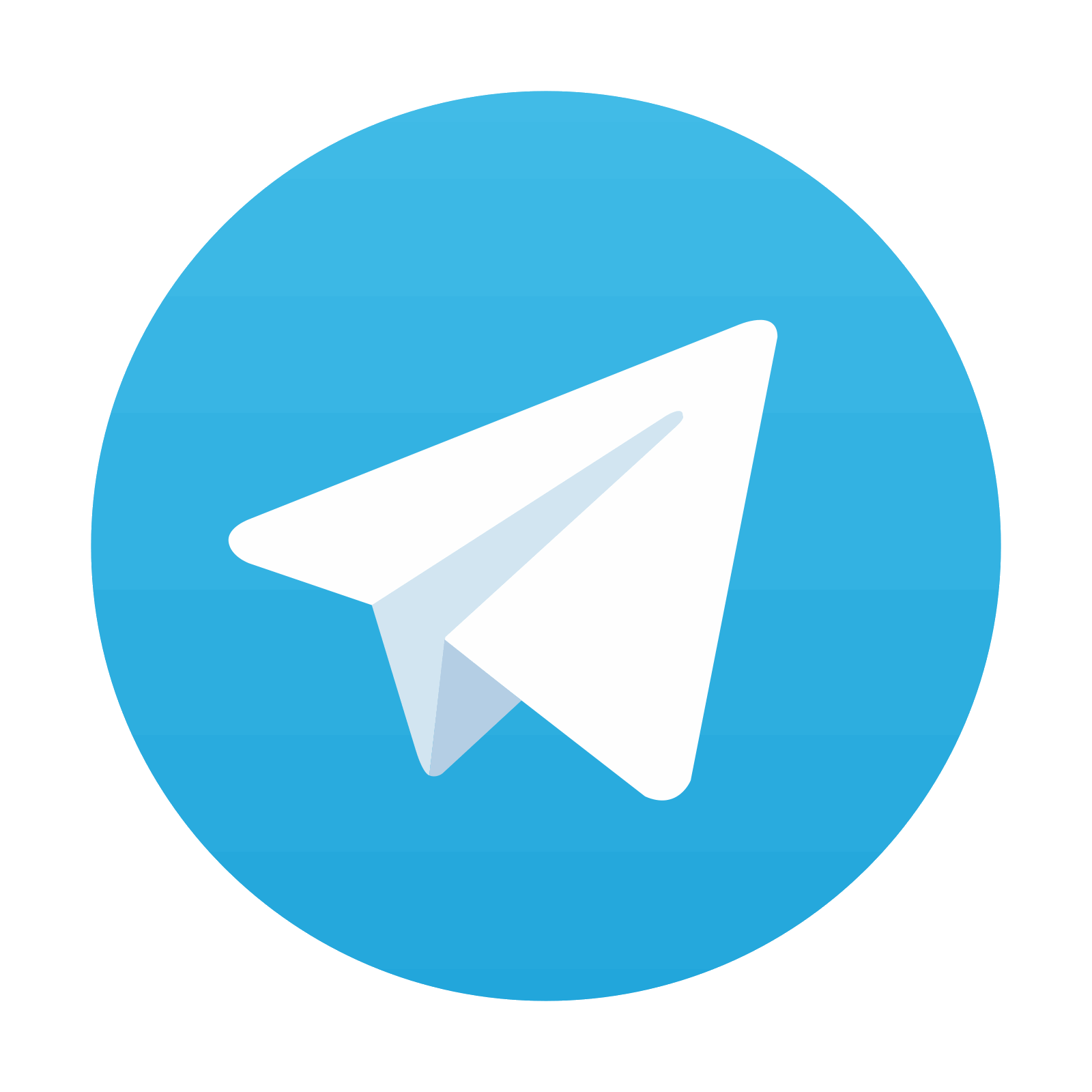
Stay updated, free articles. Join our Telegram channel
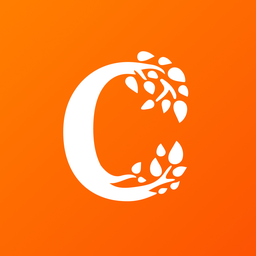
Full access? Get Clinical Tree
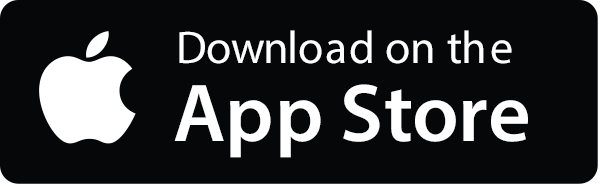
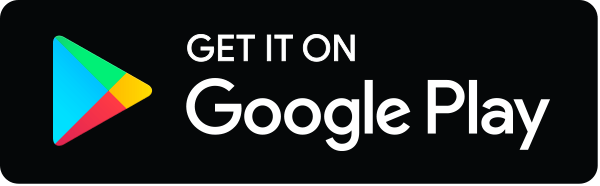
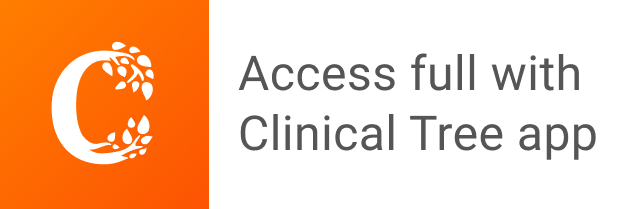