Fig. 1
Schematic of different mechanisms of ultrasound-mediated drug release, drug transport, and sonoportation from ultrasound -responsive nanoparticles
As an acoustic wave travels through a medium it is attenuated through reflection, scattering, and absorption [36]. Acoustic energy that is absorbed is converted to heat [35]. Ultrasound is thus one of the only modalities capable of generating highly localized mild hyperthermia (39–43 °C) at depth within the body. The resulting temperature rise can be monitored noninvasively using either ultrasound or MRI-based techniques, with MR-thermometry being most commonly used clinically in spite of its significant cost and limited spatiotemporal accuracy [37–40].
Ultrasonic waves are also capable of imposing mechanical effects, such as acoustic radiation force or cavitation. Acoustic radiation forces are the time averaged net force in the direction away from the ultrasound source [41]. Acoustic cavitation is the dynamic response of a gas and/or vapor cavity (i.e., a bubble) to an oscillating acoustic pressure amplitude [42]. However, bubble nucleation using ultrasound alone requires large pressure amplitudes [43]. In order to reduce the pressure amplitudes necessary for cavitation, cavitation nuclei are typically introduced via intravenous injection, and take the form of either microbubbles, also known as ultrasound contrast agents , or nanoscale cavitation nucleation agents [44, 45]. Occurrence of cavitation can be detected and monitored remotely through a technique known as passive cavitation detection , or passive acoustic mapping , whereby narrowband or broadband acoustic emissions arising from cavitating bubbles are remotely sensed [46, 47]. Acoustic cavitation has had significant impact on ultrasound based therapies [48–50] such as ultrasound-enhanced drug delivery, which is the focus of this chapter.
Non-inertial cavitation is the periodic oscillatory radial motion of a bubble, and is dependent on the size [51] and composition of the bubble [52]. This periodic motion perturbs the surrounding fluid over microsecond time scales, generating micro-streaming that results in convective transport of particles trapped in the currents [53–55], open up tight junctions between endothelial cells [56], disrupt cell membranes [57], and induce intercellular and intracellular bioeffects [58, 59]. Inertial cavitation occurs when the peak negative pressure amplitude becomes large enough to cause the bubble to unstably grow, and subsequently collapse during the positive pressure phase due to the inertia of the surrounding liquid. During the collapse phase of the bubble, jets, bubble fragments, and other asymmetric bubble shapes are often formed. The collapses emit shock waves that are detectable as broadband signals [60], which are useful for imaging techniques, such as passive acoustic mapping [61, 62]. As the collapses themselves can be periodic [63], inertial cavitation is also capable of generating micostreaming, along with the associated convective mass transport and bioeffects. As a result, inertial cavitation is a key enabler and facilitator in drug delivery.
We now describe nanoparticulate strategies that exploit the thermal and cavitational effects of ultrasound for ultrasound-triggered release, enhanced transport into biological targets, or improved delivery of therapeutics across the cellular membrane.
4 Ultrasound Triggered Drug Release from Nanoparticles
Drugs contained within biocompatible materials (e.g., lipids and polymers) to make drug-loaded nanoparticles increase disease specificity and reduce systemic toxicity of the encapsulated drug [64–67]. These drug delivery strategies are dependent on the size and composition of the drug-loaded nanoparticle [68]. However, it has also been shown that despite increased accumulation of drug-loaded nanoparticles, there is no associated increase in drug delivery within the tumor even when attempts to normalize the tumor environment are made [12, 69]. Without an external trigger to release the contents of the drug-loaded liposome, there remains a diffusive barrier of the encapsulation that prevents the bioavailability of the drug. In order to overcome these barriers, there has been a quest to develop external triggers to spatially and temporally release drugs from these nanoparticles , in order to achieve reduced systemic toxicity, enhanced drug accumulation, and improved bioavailability. Here we look at several designs of nanoparticles that release their payload in the presence of ultrasound .
4.1 Hyperthermia-Triggered Drug Release with Ultrasound
One of the key attributes for a well-designed drug delivery vehicle is incorporating a means to trigger the release of its contents. Encapsulating strategies that degrade in the presence of elevated temperatures have thus attracted considerable effort and interest. These thermosensitive drug delivery vehicles utilize heat-sensitive lyso-lipids, polymers, peptides, reactions, or a combination thereof in the shell of the vesicle (Fig. 2a). Here, we give an overview of the recent advances in thermosensitive drug delivery strategies that utilize ultrasound as the heat source.
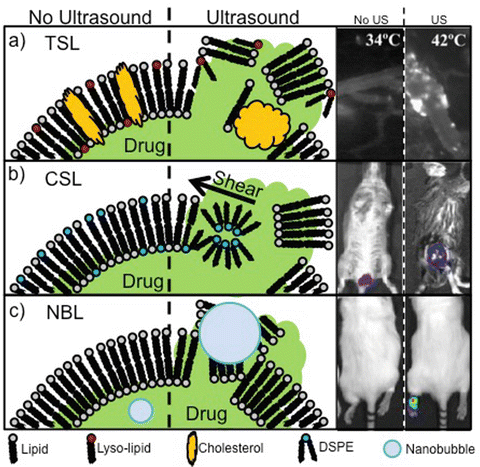
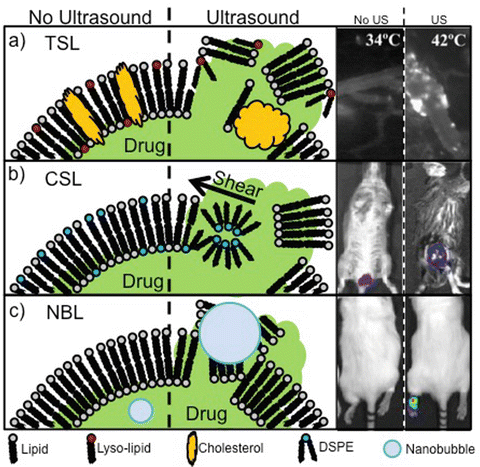
Fig. 2
Schematics and application of different ultrasound-mediated mechanisms for drug release from liposomal nanoparticles . (a) A cartoon of thermally sensitive liposomes (TSL) is shown before and after ultrasound exposure. Snapshots of blood vessels that indicate extravasation of a chemotherapeutic from thermosensitive liposomes before and after exposure to ultrasound-induced hyperthermia indicate presence of drug [74]. Image adapted with permission. (b) The mechanism of drug release from cavitation sensitive liposomes (CSL) upon exposure to ultrasound. In the presence of cavitation localized in a tumor demonstrates enhanced luciferin expression [100]. Image adapted with permission. (c) Drug release from nanobubble liposomes (NBL) is shown. Xenografted tumors in the foot of a mouse treated with NBL is shown to have increased luciferin expressions upon exposure to ultrasound [134]. Image adapted with permission
Early work on thermosensitive liposomes primarily relied on the phase transition of the encapsulating material. For example, liposomes comprised of a combination of lipids with acyl chain lengths between 16 and 18 carbons will result in a leaky membrane between 41 and 54 °C, the phase transition temperatures of the respective lipid [70, 71]. Such mild hyperthermia is easily achievable with an extracorporeal ultrasound device. However, the effectiveness of such liposomes is not evident until more severe degrees of hyperthermia (>45 °C).
A key innovation towards liposomal drug delivery was the incorporation of temperature-sensitive lyso-lipids into the lipid bilayer shell [72]. These lyso-lipids begin to break down at temperatures around 39–40 °C, achieving maximum release at 41 °C [73]. A lyso-thermosensitive liposome (LTSL) formulation has been used to encapsulate doxorubicin [74] and is now in human trials under the trade name Thermodox (Celsion, USA) [75]. Subsequently, there has been continued work to develop other formulations [76–80] as well as demonstrate the capacity to trigger release from ultrasound-induced hyperthermia [81].
Other researchers have successfully encapsulated other chemotherapeutics into a thermally sensitive liposome . For example, cisplatin (a potent therapeutic for solid tumor present in head and neck, genitourinary, and lung cancers) was encapsulated in a formulation comprised predominately of hydrogenated soybean phosphatidylcholine and cholesterol [82]. The addition of a polyethylene glycol lipid enabled the liposome to be shielded from the innate immune response [83]. Unfortunately, this particular formulation has shown poor therapeutic efficacy despite the long circulation time, which has been shown to preferentially accumulate in tumors that have leaky blood vessels [84, 85]. Schroeder et al. [86] showed that when exposed to low frequency ultrasound (20 kHz), the STEALTH liposomal cisplatin released their contents in an in vivo murine model. Though no temperature or cavitation measurements were taken, Schroeder attributed their results to the large quantities of cholesterol in the shell of the liposome. Researchers have also utilized this shell composition to encapsulate other chemotherapeutics, such as 5-fluorouracil [87].
Instead of using lyso-lipids for temperature sensitivity, other researchers have added thermosensitive polymers to encapsulate drugs [88–90]. One polymer in particular has garnered much attention owing to its sensitivity to temperatures around 40 °C. N-isopropylacrylamide (NIPAM) is a polymer that undergoes a reversible phase transition between 32 and 40 °C, depending on the polymer chain length. This phase transition changes the hydrogel structure of NIPAM to a collapsed dehydrated state, losing up to 90 % of its initial volume. As a result, this polymer allows a change in the shell morphology, opening up pores, and allowing for drug release.
An alternative to changing the properties of the liposome shell for drug release is to instead generate bubbles from within the liposome. To do so, researchers have added ammonium bicarbonate to the liposomal core [91, 92]. This chemical decomposes to form ammonia, water, and carbon dioxide bubbles at temperatures above 36 °C. However, within the liposome, researchers have shown that the temperature sensitivity of the bubble generating liposome does not occur until temperatures above 40 °C. Once a carbon dioxide bubble is generated, the sudden expansion in volume disrupts the lipid membrane. This disruption opens pores within the membrane or ruptures it entirely, releasing the payload. Furthermore, these bubbles can be imaged using ultrasound , giving a clear indication of delivery.
Another means of bubble generation from a heat source is the use of gases, such as perfluorobutane and perfluorpentane, which have relatively low boiling points. Researchers have shown that this bubble may be stored initially as a meta-stable liquid. Once a liquid droplet is formed, interfacial forces enable these chemicals to remain as a liquid in elevated temperatures (such as those inside the body) [93, 94]. Heat from an ultrasound source is capable of temporarily disrupting this equilibrium, forcing the liquid to phase change into a gas. Because many of these gases are hydrophobic, researchers have dissolved hydrophobic drugs (such as many taxane-based chemotherapeutics [95–97]) into the nanodroplets. Upon ultrasound triggered phase-change, these droplets instantaneously release the therapeutic agents into the surrounding medium.
Though there are substantial advantages of triggered drug release from heat-sensitive nanoparticles , this technology suffers from the inability to monitor heat deposition. There are currently a limited number of techniques to noninvasively monitor temperature during treatment. The methods that are currently in use (MRI guided thermometry) are slow (i.e., not in real time) and expensive. Furthermore, current thermometry techniques are fairly inaccurate. Such inaccuracies may mean the difference between no-treatment and complete treatment. Thus the key challenge in heat-sensitive technologies lies not in the nanoparticle development, but instead with techniques to monitor the success of therapy in a safe and cost-effective way.
4.2 Mechanically Triggered Drug Release with Ultrasound
In order to avoid the imaging challenges presented by heat-based drug release therapies, there has been a surge in the utilization of mechanical means to disrupt drugs encapsulated by lipids, peptides, or polymers because these can potentially be more readily monitored by ultrasound. The goal of stimulus-responsive drug carriers is to release a drug in the presence of an externally triggered event. Similar to the heat-sensitive liposomes discussed earlier, researchers have also developed a mechanical energy analogue that exploits inertial cavitation to rupture the lipid bilayer.
In order to create a lipid shell that is sensitive to cavitation shockwaves, researchers used a lipid that has the propensity to change its solid phase structure in the presence of shear forces. Distearoyl-sn-glycero-3-phosphatidylethanolamine (DSPE) forms a lamellar gel structure in ambient conditions [98, 99]. In the presence of a shockwave, the structure of the solid phase transitions from a gel to an inverted hexagon. This change in structure destabilized the lipid bilayer , allowing for an abrupt release of the encapsulated payload. Cavitation-sensitive liposomes have recently been developed to break apart in the presence of a shockwave induced by inertially cavitating bubbles [100]. In the presence of artificial cavitation nuclei (in this case SonoVue microbubbles), these liposomes achieved close to 100 % release at peak rarefactional pressures on the order 1.5 MPa at 0.5 MHz. This represents a fraction of the pressure amplitude typically required to release thermosensitive liposomes (>4 MPa at 1 MHz) and is an operating regime that is potentially achievable by conventional diagnostic ultrasound scanners rather than highly specialized and expensive high intensity focused ultrasound systems.
Cavitation-sensitive liposomes require the proximity of artificial cavitation nuclei to generate the shockwave. These cavitation nuclei can range in size from as large as 5 μm to as small as 200 nm [101], with both types of nuclei having successfully demonstrated release As a result, the source of cavitation may have different pharmacokinetics depending on the size of the cavitation nuclei. To avoid the need for secondary nanoparticles , researchers have developed echogenic liposomes.
Unlike cavitation sensitive liposomes, echogenic liposomes are hypothesized to house nanoscopic gas pockets in the hydrophobic layer, either in the lipid bilayer shell or a micelle within the liposome. Upon exposure the ultrasound , the nanobubbles reportedly cavitate and destroy the integrity of the shell. Once broken, the contents of the liposome are released. Suzuki et al. [102, 103] successfully demonstrated improved luciferase coding plasmid DNA transfection to tumors from echogenic liposomes only in the presence of ultrasound. In addition to gene transfection, echogenic liposomes have also encapsulated tissue plasminogen activator in order to improve thrombolysis therapies [104–107]. Other hydrophilic and lipophilic therapeutics have also been encapsulated by echogenic liposomes [108].
Others have reported a polymeric nanobubble with a coating of a gene-loaded micelle [109]. These nanobubbles are capable of scattering ultrasound similar to microbubbles. These nanobubbles also demonstrated sustained acoustic response greater to that of gas-core liposomes. Moreover, in vivo survival studies with murine tumor models indicated that tumor volumes treated with ultrasound and gene-loaded polymeric nanobubbles were significantly more effective at controlling or reversing tumor growth.
5 Ultrasound-Enhanced Drug Transport from Nanoparticles
One of the key limitations of many drug therapies is not only their nonspecificity (which was addressed earlier), but also their inability to access tissue far beyond blood vessels. Such a challenge exists across all drug classes and has hindered the capacity to treat diseases, such as neurological disorders and cancer . To combat this challenge, many treatments rely on elevated drug doses that often come with severe side effects. This is perhaps best reflected in cancer treatments whereby the tumor itself hinders the passage of drug beyond 20–50 μm from a blood vessel [12]. The stunted travel distance of even small drug molecules often results from the tumor physiology, despite the leaky vasculature of a tumor. Moreover, physiological barriers, such as the tight junctions of the blood–brain barrier, preclude the use of nearly any drug administered simply by intravenous injection. It is therefore crucial that new generations of nanomedicines address this concern, and focus on methods to promote not only drug specificity but also its distribution within the diseased tissue.
To address this challenge without surgical intervention, elevated drug doses, or resorting to palliative care, researchers have looked towards ultrasound as a modality to interact with deep tissue. As mentioned in the previous sections, we have shown that nanoparticles that respond to ultrasound have been implemented for site-specific drug release. Note that the methodologies to be discussed are applicable to freely circulating drugs. This is an important distinction because the encapsulation of a drug presents a substantial regulatory and financial challenge. Moreover, we want to distinguish between cellular transport (i.e., sonoporation), which is discussed in another section, and transport to extravascular tissue. Below, we show the capacity and advantages for nanoparticles to enhance the transport of therapeutics into a targeted tissue beyond blood vessels .
5.1 Improved Transport from Ultrasound -Induced Hyperthermia
There has been considerable effort in using ultrasound-induced hyperthermia to remotely trigger drug release from nanoparticles (as seen in our earlier section). Furthermore, there is evidence that hyperthermia itself enhances cell permeability, improving the efficacy of cellular drug transport [110–112]. It has been suggested that heat improves circulation in tissue with a dense microvasculature such as tumors, thereby improving local drug concentrations. However, recent work has demonstrated that hyperthermia does the opposite; blood-flow decreases due to the arteriolar–venular pressure gradient [113]. Thus, the exact mechanisms for enhanced transport of therapeutics from ultrasound-induced hyperthermia are still unclear.
Because the source of the heat is irrelevant, ultrasound provides a noninvasive means to locally increase temperatures in deep tissue, allowing for increased uptake of drugs such as monoclonal antibodies [112]. Beyond enhanced cellular uptake, it has been shown that hyperthermia from high-intensity focused ultrasound will disrupt the BBB, allowing easier passage of drugs into the brain [114, 115]. However, in these studies it was difficult to delineate the extents to which cavitation or hyperthermia disrupted the endothelial tight junctions. More recently, others [116] have shown that mild hyperthermia from ultrasound opens up the tight junctions of the BBB, allowing for increased drug uptake into the brain.
As mentioned earlier, phase-change nanodroplets have shown great promise in localized drug delivery activated by ultrasound -induced hyperthermia. Chen et al. have shown that these nanodroplets are capable of separating the tight junctions between the endothelial cells in the brain [117]. They demonstrated that there was preferential uptake of contrast agent into the brain following ultrasound exposure. Considering the beneficial effect of hyperthermia alone, it is difficult to distinguish drug transport induced by nanodroplets from hyperthermia alone.
5.2 Cavitation-Enhanced Transport of Small Molecular Drugs and Biologics with Ultrasound
Perhaps one of the most established means to promote transport of a drug beyond blood vessels is acoustic cavitation . As mentioned earlier, cavitation is typically generated by cavitation nuclei co-injected with the therapeutic . Upon exposure to ultrasound, cavitation nuclei experience large volume changes that generate microstreams and pump drugs into the tissue, as well as open up endothelial junctions (Fig. 3) [118]. To date, these cavitation nuclei are typically of the micron size range and are primarily comprised of gas (i.e., a microbubble).
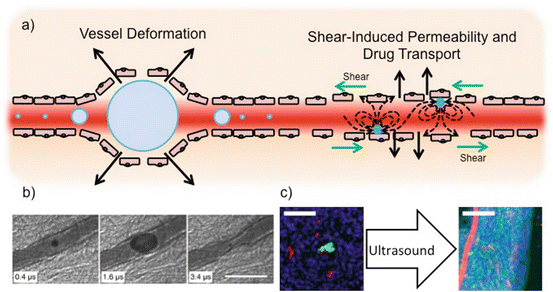
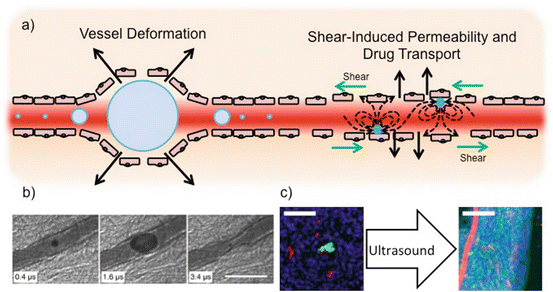
Fig. 3
(a) A cartoon of mechanical deformation and transport of a therapeutic beyond a microvessel from a cavitating bubble. Both vessel deformation and shear-induced permeability are shown (b) A microbubble inducing microbubble expansion and invagination as a direct result of cavitation in an ex vivo microvessel [118]. Image adapted with permission. The scale bar represents 50 μm. (c) Fluorescent microscopy images of a tumor treated with a co-injection of gas-stabilizing solid nanoparticles and a fluorescent antibody demonstrates the effect of ultrasound on drug-extravasation. Without ultrasound, antibody is co-localized with the blood vessel [45]. Image adapted with permission. Blue, red, and green represents the cancer cells, blood vessels, and antibody respectively
Microbubbles have long been established as the key cavitation agent used in biomedical technologies. However, many biomedical applications require submicron sizes and sustained cavitation response times. Microbubbles are suboptimal for these applications. As a result, there has been a surge in the development of submicron cavitation agents, such as nanobubbles and gas-stabilizing solid nanoparticles .
5.2.1 Gas-Stabilizing Solid Nanoparticles
Another means to generate bubbles from nanoscale nuclei is to partially stabilize a bubble on the surface of a solid nanoparticle. Much like bubbles in a champagne flute, nucleation of bubbles from solid surfaces requires defects (such as cracks and crevices on glass) that entrap gas. For bubbles on microparticles, these surface stabilized bubbles rapidly expand and detach from the surface when exposed to shockwaves. The expulsed bubble pushes the microparticle away, actively propelling it away from the cavitation site. This phenomenon has been well established on micron size particles, but there has been limited number of studies for gas-stabilizing nanoparticles , especially in the context of drug delivery .
Creating surface defects on nanoparticles capable of trapping gas is an immense challenge. Borkent et al. [119] have shown that a single well-defined cavity is able to trap a nanobubble . Upon exposure to a shockwave, the bubbles trapped within these nanopits expanded and detached from the cavity. However, surfaces such as those are impractical for drug delivery applications. Single “cup” shaped cavities that trap gas are nevertheless possible on nanoparticles [45]. Moreover, the cavities on these “nanocups” are tuneable [120]. Much like the nanopits on the surfaces presented by Borkent et al. [119], these nanocups are able to eject a cavitating bubble from their cavity. Once ejected, these cavitation bubbles rapidly expand and collapse, emitting a broadband signal indicative of inertial cavitation. The inertially cavitating bubble has been shown to promote drug delivery in both in vivo and in vitro experiments [45]. Because these particles exclusively emit broadband emissions, they are detectable with diagnostic ultrasound probes.
Others have also developed gas-stabilizing nanoparticles . In contrast to the nanocups, these nanoparticles contain gas within the pores of the nanoparticles. When exposed to ultrasound, gas from within the pores extends out, nucleating a cavitating bubble. Studies have shown that such cavitating bubbles are capable for diagnostic ultrasound [121]. However, there has not been any study that has evaluated the ability for these nanoparticles to promote drug transport.
In principle, these nanoparticles simply provide a source of nanobubbles . It is these bubbles that provide the means by which a circulating therapeutic extravasates beyond the blood vessel. The mechanism of action for enhanced extravasation is mechanical in nature, and as such is drug-class-agnostic. As a result, the key advantage of cavitation, inducing solid nanoparticles , is their capacity to promote the effectiveness of therapies across several clinical indications without the need to modify existing drugs.
6 Sonoporation
Gene therapies require the delivery of genes to the nucleus of the cell. However, there are substantial challenges in promoting cellular uptake of these genes. Sonoporation, therefore, is the use of ultrasound to temporarily permeate the cell membrane wall, allowing nucleic acid polymers (DNA, RNA, siRNA, etc.) to enter the cytoplasm.
Mechanistically speaking, sonoporation occurs from cavitation [122, 123]. Cavitating bubbles, as we discussed earlier, enable highly localized shear forces with shear rates on the 107 s-1 [124]. Such shear rates near the cell wall force the cell membrane to temporarily open. Alternatively, shock waves generated by collapsing bubbles are also capable of disrupting the cell membrane wall. In addition to shock waves, jets formed by an inertial cavitation bubble [125] have also been shown to temporarily form pores on the surfaces of cells [126, 127]. These temporary openings in conjunction with the enhanced transport associated with cavitation results in an effective means to transfect diseased cells.
6.1 Sonoporation from Ultrasound Induced Hyperthermia
Earlier in sections 4 and 5, we discussed the use of phase-change nanodroplets for both drug release and drug transport. It is therefore not surprising that these nanodroplets have also been used for gene delivery. For example, Burgess and Porter [128] demonstrated successful transfection of cancer cells. To do so, they utilized a green fluorescent protein expressing siRNA freely suspended with phase-change nanodroplets. Expression of GFP only occurred in the presence of high intensity focused ultrasound (5 MHz center frequency at 6.2 MPa). Others were able to bind DNA to phase-change droplets. Upon exposure to ultrasound and DNA bound to phase-change droplets, Gao et al. [129] demonstrated a substantial increase in transfection of HepG2 cells.
Other temperature-sensitive materials (such as poly-NIPAM) have also shown promise for gene therapy [130]. However, there have been few (if any) studies that have manufactured nanoparticles with these materials and applied ultrasound induced hyperthermia for cell transfection .
6.2 Mechanically Induced Sonoporation by Ultrasound -Mediated Cavitation
A more direct route to permeate the cell membrane is to utilize the innate mechanical responses of pre-formed bubbles (Fig. 4). As of late there has been a surge of interest in the development of nanobubbles. These nanobubbles either exist as a stand-alone gas bubble with various coatings [131, 132] or are encapsulated within a hydrophobic shell or are contained within a liposome [133]. In our earlier sections, we discussed the benefit of several of these nanobubble constructs for ultrasound -mediated drug release. Here we focus on their capacity to also promote cell transfection.
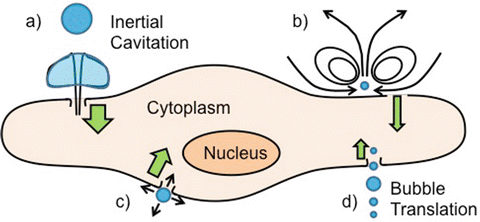
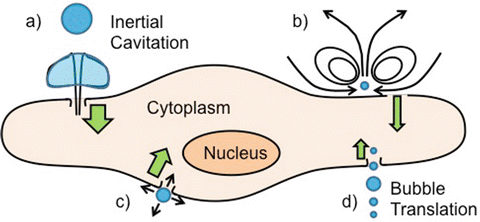
Fig. 4
A diagram of different mechanisms by which a cavitation bubble from an ultrasound -responsive nanoparticle can enhance permeation of a cell membrane and promote gene transfection is shown through (a) inertial cavitation, (b) microstreaming and micropumping, (c) membrane deformations, and (d) bubble translation as a result of acoustic radiation force
As already discussed, hydrophobic gases have been shown to preferentially reside in the hydrophobic regions of a lipid bilayer encapsulation or within a liposome encapsulation. Upon exposure to ultrasound, researchers have shown that the radial oscillations of the nanobubble will disrupt the lipid membrane, thereby releasing its payload. Thus, these echogenic liposomes have shown great promise in drug release. Beyond their capacity to release drugs, the cavitating nanobubbles also generate mechanical forces that affect the surrounding medium. For example, echogenic liposomes have been shown to improve gene transfection into cancer cells in a tumor, which requires the permeation of the cell membrane [102, 134–136].
7 Conclusion
In this chapter, we review the use of ultrasound -responsive nanoparticles , which cause either thermal or mechanical effects, to address the key clinical challenges of current drug therapies, and in particular (1) the triggered release of a drug from a nanoparticle, (2) the extravasation and enhanced transport of a free or recently released drug to diseased tissue, and (3) the enhanced cellular uptake of a gene therapy or other pharmacological agent.
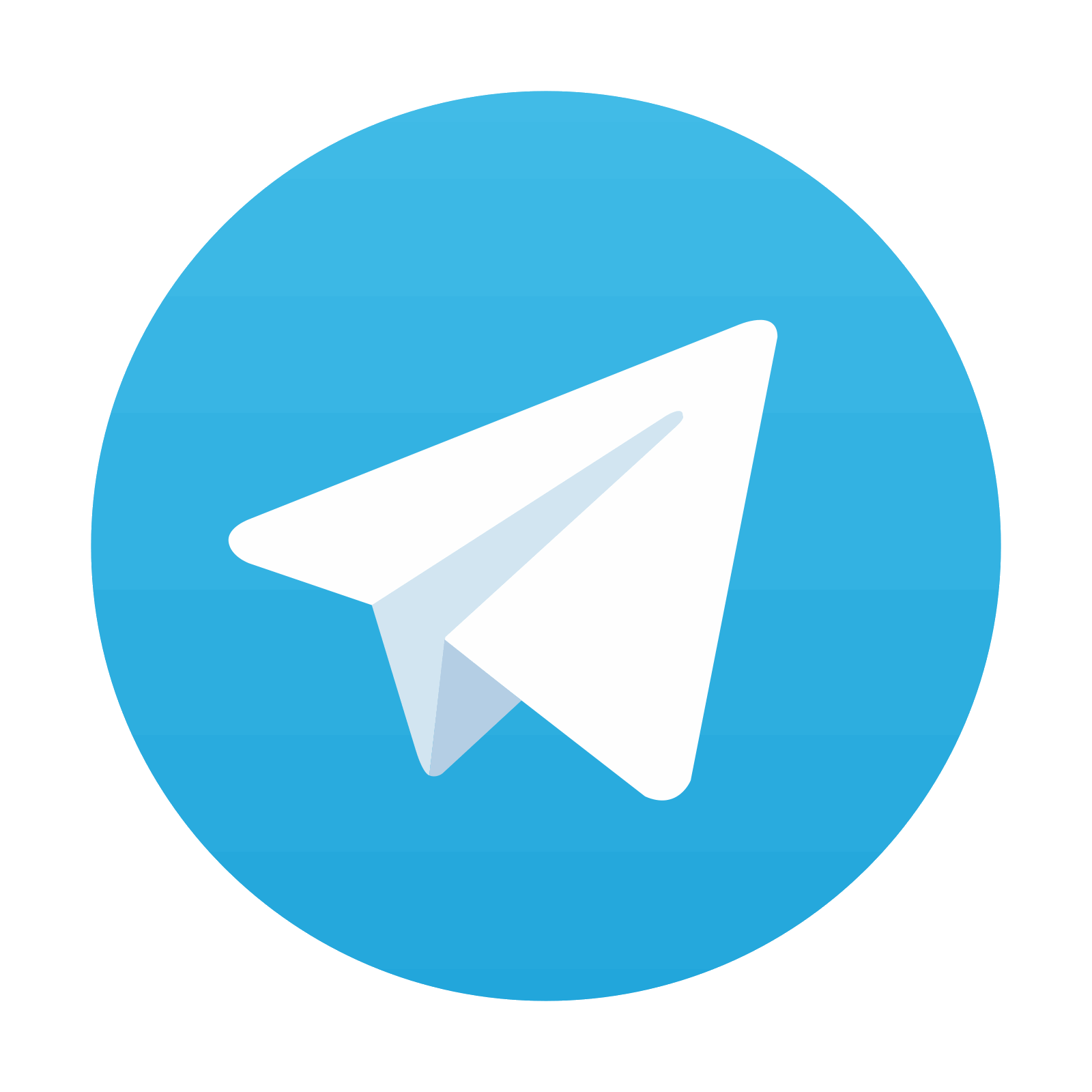
Stay updated, free articles. Join our Telegram channel
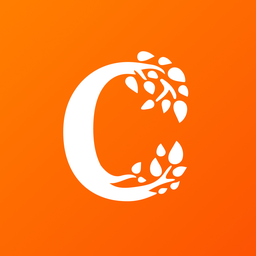
Full access? Get Clinical Tree
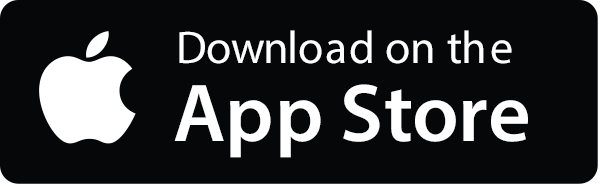
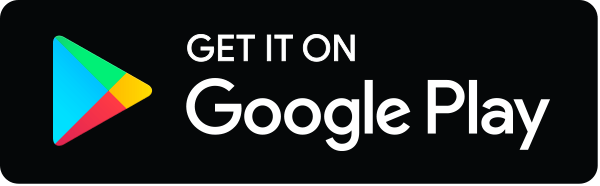