Fig. 1
Double-disk magnetic structure and field diagrams. (a) Schematic of the field (small black arrows) from two parallel disks magnetized to saturation by the background field of an MRI scanner (large red arrow). Nonmagnetic spacer elements are omitted for clarity. (b) Calculated (negative) field magnitude in the mid-plane through a typical magnetized disk set, contrasting its homogeneous nature between the disks with its rapid external decay (reproduced in part from [101], with permission, Nature Publishing Group)
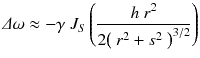
Double-disks are not the only structures that can frequency-shift the NMR signal. As mentioned, the main requirement is generating a uniform offset field region and this can be done in more than one way. For example, short, hollow cylindrical tubes with a length approximately equal to their diameter can also generate the necessary water-accessible, spatially extended regions of uniform field offset within their interior [126]. Figure 2 shows an example of the fields of such a structure which give frequency shifts of [126]
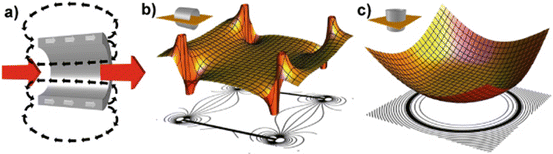
Here, γ and J S are defined as previously but now L represents the tube length, 2ρ is its diameter, and t is its wall thickness. Again for simplicity a thin-walled structure with t < < L ≈ 2ρ has been assumed. Analogously to the double-disk structures, the field magnitude can be controlled by changing the tube length, the tube diameter, the tube wall thickness, or the magnetic material from which the tubes are made.
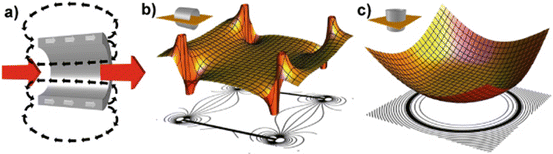
Fig. 2
Schematic of the fields generated by a magnetized hollow cylinder. (a) Cut-away schematic of the field (black arrows) of a hollow cylinder magnetized to saturation by that background MRI field (larger red arrows). (b) Calculated magnetic field magnitude profile with underlying field magnitude contour plot in a mid-plane through a magnetized hollow cylinder. Plane orientation shown in upper left corner. (c) As for (b) but for perpendicularly oriented mid-plane (reproduced in part from [126], with permission, IOP Publishing)
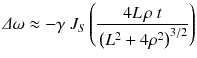
Interestingly, uniform field regions can also be created by asymmetric structures. An example of this is open elliptical shell structures that have their inner and outer boundaries defined by ellipsoids of differing eccentricities [127]. As surfaces of second degree, ellipsoids offer truly uniform internal magnetizations [128]. For magnetically saturated ellipsoids, such uniform magnetization can be shown to result also for geometries that represent one ellipsoidal volume removed from within another, no matter whether the ellipsoidal volumes share a common center [127]. Thus various counterintuitive asymmetrical structures become possible, an example schematic being shown in Fig. 3. For these elliptical shell agents, the internal uniform field magnitudes and resulting frequency shifts depend on the difference in aspect ratios, or eccentricities, of the ellipsoidal shapes that define the structures’ physical boundaries. A relatively simple case is a hollow shell structure formed by subtracting a spherical volume (of any radius r) from within an ellipsoid of revolution with semi-axes r(1 + ε a) and r(1 + ε b); frequency shifts are described by [127]
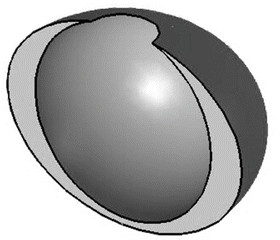
where for simplicity again, a thin shell structure has been chosen with ε a and ε b much less than unity. Here, field magnitudes and NMR frequency shifts can be controlled by changing boundary ellipticities, by changing the size of either outer or inner bounding ellipsoid (thus changing shell “thickness”), or by changing the magnetic material from which the ellipsoidal shell is constructed.
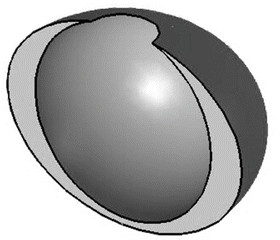
Fig. 3
Schematic of open, asymmetric ellipsoidal shell structure. Cross section through an open shell geometry defined by an oblate ellipsoid of revolution (oblate spheroid) after a vertically offset spherical volume has been removed
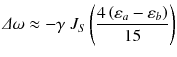
As magnetic particles, all of these structures double as T2 contrast agents . Indeed, one can imagine transforming a T2 agent into a multispectral agent simply by redistributing its material, reforming the spherical (or randomly shaped) particle into a double-disk, hollow cylinder, elliptical shell, or any other shape capable of generating the necessary magnetic field profiles. No new magnetic material needs to be added or removed in the process. Since microparticle T2 relaxation is dominated by transverse dephasing in the far field where particle shape is irrelevant, a multispectral microparticle agent created in this way would possess the same relaxing ability of the original microparticle agent. But by adding a local uniform field region, the material redistribution adds shape-identifying spectral information that enables particles that would otherwise appear identical in an MRI scan to be distinguished from one another.
Such particles therefore make interesting candidate labels for MRI-based cell tracking, a growing MRI application that may be of considerable value to new cell-based medical therapies [129, 130]. If surface functionalized such that different microstructure geometries target different cell types, labeled cells could be tracked using regular T2*-weighted gradient echo imaging protocols, but also distinguished from one another through the geometrically encoded spectral content of their magnetic labels. Added spectral content could also distinguish hypointense image regions due to the administered contrast agent from those due to natural image darkenings or signal voids arising from air bubbles or other magnetic field inhomogeneities. Such “color” particle distinction can be seen in Fig. 4, which shows different resulting frequency shifts from double-disk structures with different disk thicknesses .
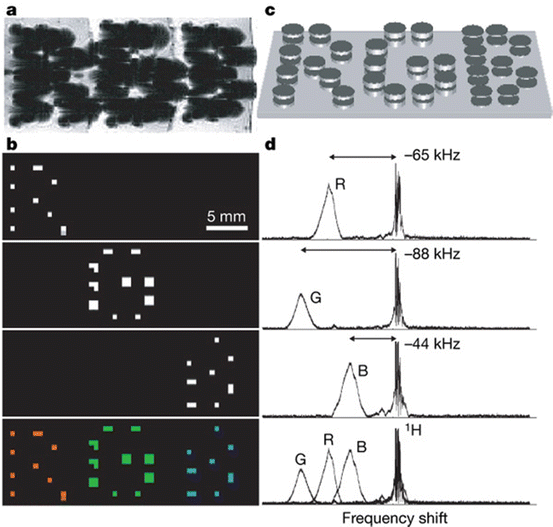
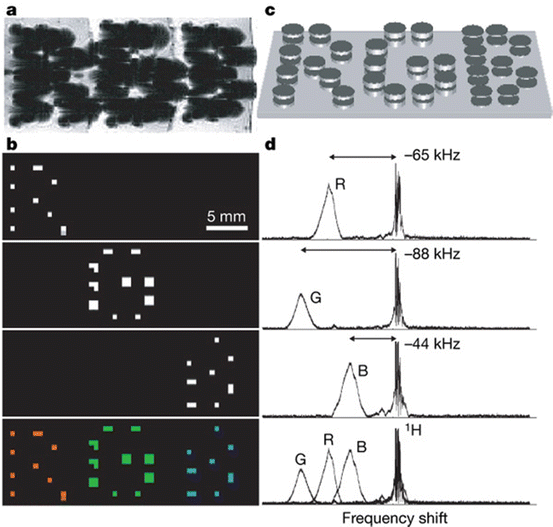
Fig. 4
Multispectral MRI. (a–d) Chemical shift imaging of demonstration 1.25 mm diameter particles magnetized by background MRI field. Particle frequency was varied by changing the thickness of electroplated nickel layers that formed the magnetizable disk pairs. As with normal magnetic particle detection, magnetic dephasing due to the particles’ external fields enables the spatial imaging shown in the gradient-echo MRI (a). However, comparison between (a) and the chemical-shift images (b) shows that the additional spectral information both differentiates between particle types and improves particle localization. The particles are shown schematically (not to scale) in (c). With particle spectra (d), to the right of the corresponding chemical-shift images in (b) shifted well clear of the water proton line, different planes in the chemical-shift imaging map isolate different particle types for unambiguous color coding with minimal background interference (b, bottom panel). (Although still visible in the gradient-echo image, the top-corner particle of the letter “B” was damaged, causing its shifted frequency peak to vanish) (reproduced from [101], with permission, Nature Publishing Group)
4.3 Frequency-Shifting Properties
Figure 5 displays several scanning electron micrograph (SEM) images of microfabricated magnetic double-disk, hollow cylinder, and ellipsoidal shell sample structures. Although geometrically distinct from one another, the shapes are unified through their frequency shifts that all reduce to
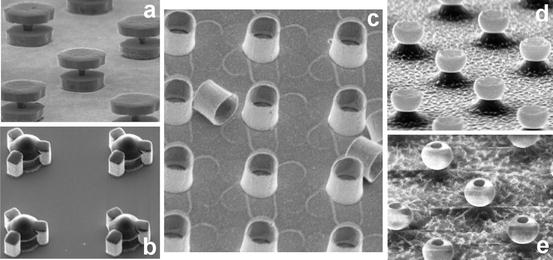
where X represents the bracketed portions in the equations above. As can be seen, in all cases X is a dimensionless function of solely the structure geometry. That is, at least as far as the magnetics are concerned (although not necessarily as far as the dynamics are concerned), shape-based frequency shifting is in principle scale invariant. Isotropically expanding or contracting any of the structures does not change the frequency shift, allowing agents to be produced over a large size range for different potential applications. Being independent of any chemical exchange processes and with material dependences appearing only through the gyromagnetic ratio and magnetic saturation polarization density, structures can also be used with any NMR-active medium and made from any magnetic material. This allows agents to be made from less toxic materials than the lanthanide ions used in PARACEST and clinical T1 agents, which require powerful chelating ligands to protect the body from direct exposure [131]. To date, shaped particle agents have been made from nickel [101, 126, 127] as well as from more biocompatible materials including iron [132] and iron oxides [133]. They have also been shown to operate equally in water and in deuterium oxide [101], and have been produced with sizes ranging from milli- to micro- to nanoscale [101, 126, 134].
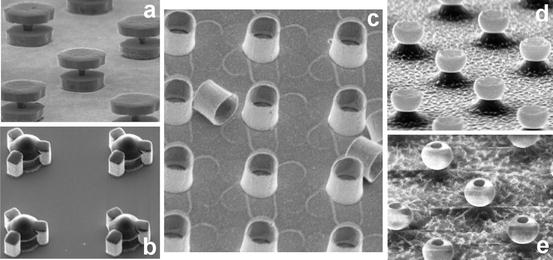
Fig. 5
Scanning electron micrographs (SEM) of microfabricated contrast agent microstructures. (a, b) SEM of magnetic double-disk structures separated by nonmagnetic internal or external spacing posts, respectively. (c) SEM of hollow magnetic cylinders. (d, e) SEM of open oblate and prolate ellipsoidal magnetic shells, respectively. For scale, all structures are a few micrometer in total size
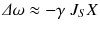
Lacking spherical symmetry, different particle orientations relative to the applied field can lead to different surrounding magnetic field profiles. These would change the resulting field shifts, were it not for the particles’ self-aligning properties. Much like a compass needle in the earth’s field, the particles’ magnetic shape anisotropy causes strong magnetic torques that automatically align the particles parallel to one another when placed into the large fields of an MRI scanner [101]. This avoids random spread in resulting resonance shifts that might otherwise hinder the ability to distinguish different particle geometries.
Because particles can be made with ferromagnetic materials, which have far greater magnetic permeabilities than para- or diamagnetic materials, the range of accessible frequency shifts is large. Iron has a J S value of over 2 T, for example, allowing NMR shifts for water protons to be engineered anywhere from zero up to tens of MHz. (In theory, shifting up to 100 MHz is possible, albeit only for unwieldly structures with very thick magnetic layers.) But even at 1 MHz or a fraction thereof, shifts easily exceed anything yet achieved with molecular agents.
Unlike paramagnetic (or diamagnetic) CEST agents, shifts generated by microparticle structures can also be field independent because typical MRI field strengths magnetically saturate the constituent ferromagnetic materials. Large field-independent shifts are advantageous because they raise the possibility of using such agents at clinical field strengths, rather than at the higher field strengths used to increase the shifts of molecular based (PARA)CEST agents. Whereas most chemical shifts are proportional to the MRI field and thus reported in relative terms of parts per million (ppm), for these shaped particle agents NMR shifts are absolute. In conventional, relative terms their shifts will therefore appear to change based on the applied field but, to aid comparison, for a 1.5 T clinical field strength MRI scanner, a MHz shift corresponds to around 15,000 ppm. Chemical shifts in NMR spectroscopy, by contrast, typically measure just a few ppm. Frequency shifting far from the background bulk water relaxes bandwidth constraints on the off-resonance radiation pulses used to address these agents. With little chance of any RF power leaking over to excite the background water, it enables virtually background-free imaging. That is, even though the signal still comes from water surrounding the magnetic particle rather than from the particle itself, the large shifts enable a form of “hotspot” imaging [135], different in mechanism, but not unlike that reported with highly shifted proton (HSP) MR imaging [136] or with background-free imaging of 19F labels. Based on magnetic nano- or microparticles that interact with surrounding water, these agents do however offer higher sensitivity than HSP or 19F imaging, in large part due to their large frequency shifts that also enable significant signal amplification.
4.4 Diffusion-Driven Signal Amplification
In some cases, the frequency-shifted signals encoded by shaped magnetic nano- and microstructures can be directly imaged via chemical-shift imaging, a standard MRI protocol that spatially maps NMR frequencies. An instance of this was already shown in Fig. 4. Just like any other magnetic particles the shaped structures appear indistinguishable in a T2-weighted gradient echo image. But in a chemical-shift image they are clearly distinguished from one another (as well as from the background water) through their ability to locally frequency-shift the nearby water by different amounts. Also clear, however, is that the frequency-shifted signals cover smaller areas than do the T2 contrast signals. This is because the frequency shifting occurs within the particles’ homogeneous field regions, which are small compared to the far-field volume over which water is transversely dephased. This means that shaped particles can be better spatially localized through their added spectral content, but it also means that their spectrally distinguishing signals are not as strong as their associated T2 contrast signals.
To boost spectral signals, therefore, a variation of magnetization transfer imaging [137] is used, not unlike that employed for amplifying CEST-based signals. Here though, proton exchange does not imply chemical exchange. Instead exchange is driven by water self-diffusion that randomly moves water molecules into and out of the homogeneous field region. The particles’ open structure design exploits this natural water diffusion to continually refresh protons contained with the field-shifted region. This yields an effective water volume from which signal can be acquired that may be several orders of magnitude larger than the homogeneous field region itself. That is, signal can be acquired not just from water that happens to be in the field-shifted region at one point in time, but from water passing through that region over an extended time period (of order the bulk-water longitudinal relaxation time, T1).
The signal acquisition protocol is similar to that of CEST agents. A series of pre-saturating RF pulses, applied at a specific offset frequency from the background water resonance, are followed by a single on-resonance pulse and the resulting free induction decay (FID) signal used to infer the resulting reduction in the bulk water signal. This process can then be repeated for different offset frequencies, building up a z-spectrum that reveals the particle-induced shifted resonance through a spectrally localized dip in the remaining water signal. (Examples of such NMR z-spectra can be found later in Fig. 6.) Alternatively, knowing contrast particles’ specific offset resonance frequencies allows for selectively addressable contrast that can be turned off and on.
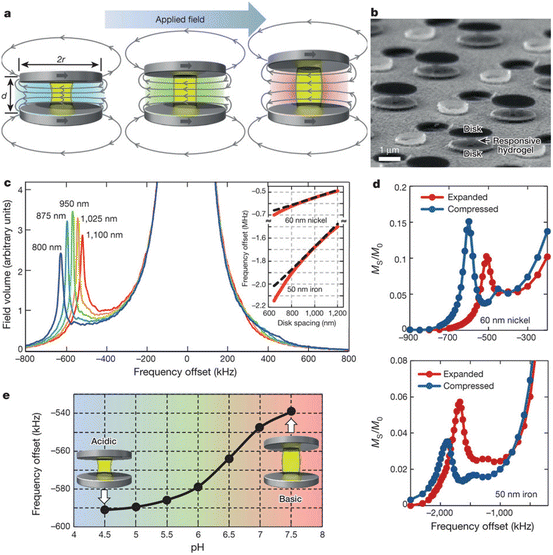
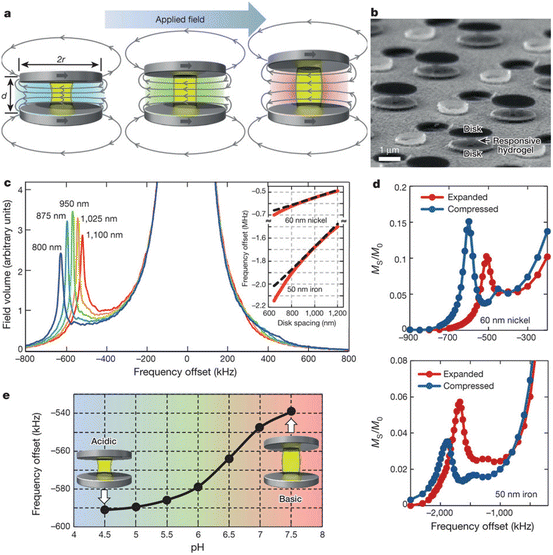
Fig. 6
Principles of shape-changing RF colorimetric sensors. (a) Schematic of sensor assemblies comprising two parallel disks magnetized by applied MRI field (blue arrow) and separated by stimuli-responsive hydrogel spacers (yellow). Resulting magnetic fields (grey curves) are uniform between the disks and locally shifted NMR frequencies of water passing through proportionally to the field magnitude, which depends on disk spacing d. Different frequency shifts represent different effective RF “colors.” (b) Scanning electron micrograph of sensors. (Interspersed features are nonmagnetic residual topography from the microfabrication process.) (c) Theoretical precession frequency (or equivalently, field) histograms, mimicking NMR spectra, for 60 nm thick, 1 μm radius nickel disk pairs with disk spacing indicated. Background water appears at zero offset; shifted peaks result from uniform field regions between the disks. Inset: frequency offset versus disk spacing for nickel and iron disks with thicknesses shown. Dashed black curves are analytic approximations (see equation (1) in ref. [132]); red curves result from numerical field simulations. (d) Experimental NMR z-spectra for nickel (top) and iron (bottom) sensors with hydrogel spacers in compressed and expanded states. Magnetization saturated out M S is normalized to the initial water magnetization M 0. (e) Experimental pH-dependent NMR shifts for nickel-based sensors containing pH-sensitive hydrogel spacers designed to shrink (expand) at low (high) pH with peak sensitivity in the physiological pH range (reproduced from [132], with permission, Nature Publishing Group)
Where shaped-particle signal amplification differs from that of molecular-based chemical exchange is in the number of, and the rate at which, protons that can be exchanged. Unlike chemical exchange processes, in which only a fraction of a molecule’s protons are able to participate, with shaped particles most of the uniformly field-shifted volume of water can be exchanged simultaneously. Exchange rates are also no longer dependent on chemical rate constants; they depend on the time it takes water to self-diffuse through the particle’s field-shifted region. Since diffusion distances scale with the square root of time, the exchange rate speeds up quadratically as particle sizes shrink, increasing the total signal that can be acquired. That is, while frequency shifts are independent of overall particle size, diffusion-driven signal amplification favors smaller (and less biologically invasive) particles.
Increasing exchange rates do increasingly broaden the shifted resonance linewidth. As mentioned with regard to CEST imaging, for agents to be effective, this broadening, which is proportional to the exchange rate, should not become so large that the shifted resonance overlaps with the unshifted background water [93]. Being ferromagnetic, particle agents offer larger frequency shifts than paramagnetic molecules, thus allowing higher exchange rates, which increase signal and should allow particles to be scaled down to below 100 nm in size. Taken together, shaped particles allow more protons to be simultaneously exchanged and allow those exchanges to recur more rapidly, yielding signal amplification and resulting contrast agent sensitivities that compare favorably with commonly used clinical T1 agents [132].
5 Geometrically Based MRI Sensing
Just as optically based fluorescent tags and labels were soon followed by fluorescent sensors, MRI contrast agents have since expanded to include responsive MRI probes [138]. Such agents change surrounding image contrast in response to some chosen biomarker, which may include various biologically significant metal ions, biomolecules, or surrounding environmental conditions such as temperature or pH. Examples include changes in T1 due to modified water access to the Gd ions [139], switches in T2 due to induced aggregation of magnetic nanoparticles [78], changes in CEST contrast due to modified proton exchange rates [140], and signal switching in shaped particle structures by (un)blocking their homogeneous shifted field regions [101].
Whether based on T1, T2, CEST, or shaped particles, these sensing examples all amount to changes in the amplitude of the contrast agent signal. As mentioned, however, such signal changes cannot necessarily be distinguished from more mundane changes in the contrast agent concentration. For in vitro tests, concentrations may be well controlled, but this is not always true for in vivo studies where precise trafficking and pharmacokinetics of the administered contrast agents may be poorly known. An option is to administer two different contrast agents [141]. By assuming that at least the ratio of their concentrations remains constant, unwanted concentration dependences can be eliminated. But such ratiometric correction may require larger overall amounts of exogenous agent and may still fail if there is any difference in agent pharmacokinetics.
One way to avoid ambiguity is through a responsive probe that reports via changes in NMR signal frequency rather than amplitude. Unlike signal amplitudes, NMR frequencies need not depend on contrast agent concentrations, allowing for more quantitative measurements. With resonant frequency shifts that can be directly engineered through particle geometry, multispectral shaped-particle contrast agents are well suited to the task. Converting such contrast agents into sensing agents is conceptually simple, requiring only a modified structure whose shape is no longer static, but can dynamically vary in an appropriate way in response to the chosen biomarker. As the structure changes shape, its surrounding magnetic field profile changes, in turn changing the local frequency shift imparted on the surrounding water signal. Given the large frequency shifts of shaped-particle agents, resulting responsive changes in those frequency shifts can be similarly large, in principle enabling even small biomarker changes to be detected.
The first such shape-changing, frequency-based, MRI sensor agents have only recently been published [132]. Referred to as Geometrically Encoded Magnetic (GEM) sensors , they employ stimulus-responsive polymer gels that effect the necessary shape changes and that enable continuous and reversible operation. (Interestingly, Paul Lauterbur , one of the original inventors of MRI, first proposed using a gel-based agent over two decades ago [142]. Except, without specific control over their magnetic fields, those agents yielded particle aggregation-dependent changes in relaxation, or signal amplitude, much like relaxation switch sensors [78].) The first examples of sensors that controllably change fields and therefore frequencies, however, used acid-sensitized hydrogels [143] to transduce local pH levels into NMR-readable frequency shifts. These sensors borrowed from the double-disk multispectral MRI agent geometry, using this time nanoscopic hydrogel pillars as spacing elements between the two magnetic disks. As these spacers swell or shrink in response to the pH of the surrounding solution, the attached disks move further apart or closer together. The resulting change in magnetic field magnitude between the disks then manifests as a changed offset resonance frequency for water passing between the disks, as detailed in Fig. 6. Since hydrogels can expand or contract by large amounts and since frequency changes can be shown to be proportional to length changes in the hydrogels separating the disks [132], large stimulus-induced spectral shifts are possible. Although stimuli-reponsive hydrogels, which rely on solute diffusion through the gel, offer notoriously slow macroscopic response times, for the nanoscopic hydrogel elements incorporated into the GEM sensors diffusion times are rapid, enabling sub-second response times.
As their name suggests, GEM sensors depend on geometry. Like the originating multispectral shaped-particle MRI contrast agents , GEM sensor signals are an intrinsic function of the shapes of the nanostructures involved. They afford not just another example of how particle shape enables new functionality, but of how the shape itself can function as a reporter of local conditions, which may extend beyond pH to include reporting on many other conditions and/or biomolecules of interest. Many different hydrogel formulations—many of them biocompatible—have already been developed in other fields and there exists considerable literature demonstrating the ability to sensitize them to many variables of interest [144]. (Nor are hydrogels the only possible polymers that could be used to effect the necessary shape changes.) Gel sensitization techniques include molecular imprinting of gels [145], the incorporation of catalytic enzymes [146] or enzyme-cleavable substrates [147], and the inclusion of specific receptor-ligand-type recognition bondings [148]. By using hydrogels with responses tailored to different targets, the same geometrically based sensing platform should be adaptable to measure a variety of biomarkers. The multispectral nature of these agents suggests also that multiple different biomarkers might be measured simultaneously by using multiple different GEM sensors . Provided that these sensors are engineered with different initial frequency offsets, different sensor signals can be spectrally isolated from one another even if spatially co-located. This may allow for different sensors to be calibrated against each other and/or for panels of biomarkers to be measured simultaneously to better discriminate between pathologies.
6 Particle Synthesis
A distinguishing feature of shaped particle agents is their synthesis. MRI contrast agents have always been produced by bottom-up chemical synthesis routes; shaped agents have instead leveraged top-down microfabrication techniques [149]. They are produced using the same technology that underpins the electronics revolution and the ever-present, but ever-shrinking, integrated circuit. Supported by an indomitable semiconductor industry, investment in micro- and nanofabrication research over many years has resulted in powerful sets of tools, able to pattern materials with features that are now reaching less than a hundred atoms on a side. Although not used before for contrast agent synthesis, the extraordinary control that such tools offer over both structure shape and composition makes them a good choice for a contrast agent whose function depends on its geometry and material makeup. Of course, an MRI contrast agent is quite different from an integrated circuit and their microfabrication does require adaptation of traditional microfabrication protocols, but several routes have already been proven [126, 127, 132, 133, 150].
Top-down fabrication is not without its limitations, however. As the billions of transistors in each of today’s billions of smartphones abundantly prove, top-down microfabrication is well suited to the creation of very small, very precise structures in a massively parallel manner. But throughput still cannot match that possible through bottom-up chemical synthesis, which may lack the precision, but which can produce large volumes of particles at a time. And even though resulting microfabricated components need not be expensive, the necessary microfabrication tools themselves are sometimes out of reach of academic labs, limiting the number of researchers that might otherwise be able to further develop particle-based multispectral contrast agent technology. Ironically then, while microfabrication is a key, enabling technology for proof-of-principle demonstrations of new structures and new functionalities, a current handicap of such structures may be precisely their dependence on such technology.
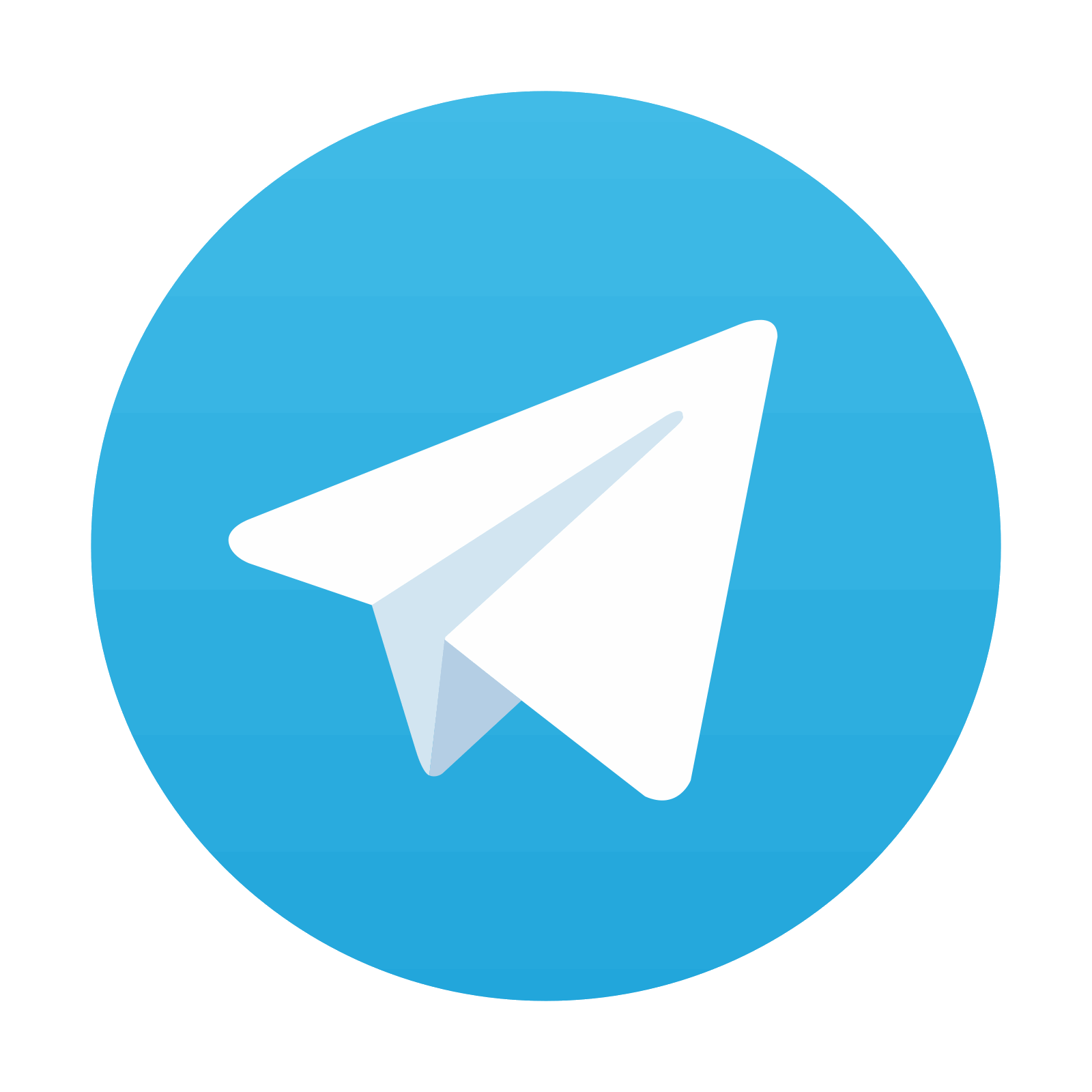
Stay updated, free articles. Join our Telegram channel
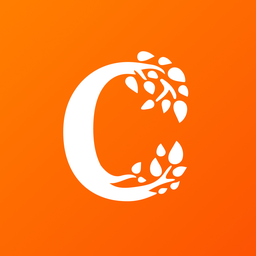
Full access? Get Clinical Tree
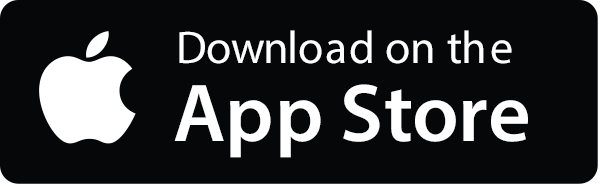
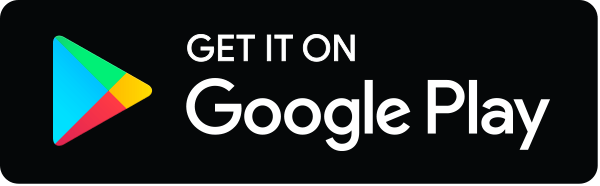