Fig. 10.1
Metric optimized gating. A synthetic trigger with longer R-R interval is used to acquire the k-space data. Hypothetic trigger locations are then retrospectively applied to the data and iteratively reconstructed with the correct average R-R interval identified as the reconstruction with the least image artifact
A second important milestone in the evolution of fetal CMR has been the recognition that information about fetal oxygenation might be obtained from taking advantage of the different magnetic properties of diamagnetic oxygenated hemoglobin and paramagnetic deoxyhemoglobin. This effect results in a shorter T2 and T2* of blood samples containing more deoxygenated hemoglobin. Sorensen et al. have used blood oxygen-dependent imaging (BOLD) to detect changes in T2* in various fetal organs in response to changes in the inspired oxygen content of the pregnant ewe and human [49]. A similar approach has been used by Wedergartner et al., who have also shown the feasibility of making measurements of the T2 of blood in the cardiac ventricles of fetal lambs as the basis of a fetal oximetry technique [56].
10.3 Fetal MR Oximetry
The Luz-Meiboom model defines the physical basis for the relationship between the relaxation of the MRI signal and the proportion of hemoglobin in blood that is bound to oxygen [25]. Wright et al. derived a term that relates T2 to oxygen saturation (SaO2) [58]:
where T2o is the T2 of fully oxygenated blood for a given hematocrit and K is a constant that is dependent on the magnetic field strength and the refocusing interval of the T2 preparation sequence used to measure T2. We have shown good agreement between MR oximetry and conventional blood gas measurements made in the large mediastinal vessels of children undergoing cardiac catheterization [33]. Since this work, modern sequences have been developed for performing MR relaxometry that produce maps allowing ready placement of a region of interest (ROI) within the vessel lumen for measuring the T2 of blood. Stainsby et al. have provided guidance on the requisite conditions for avoiding contamination of the signal from structures surrounding the vessel, which include a minimum of six voxels across the diameter and a slice thickness no greater than the vessel diameter [51]. This poses a challenge to imaging fetal vessels because shorter scan times are required to avoid excessive motion artifact, resulting in the usual trade-off between signal-to-noise ratio and spatial resolution. However, innovations in sequence design have helped to make the technique feasible in the larger vessels of late gestation fetuses. One such innovation is a sequence designed for myocardial relaxometry that employs a rapid SSFP readout following the T2 preparation and a nonrigid registration motion correction algorithm [12, 13]. The fast readout provides individual T2 preparation images that are free from motion artifact. However, it is only with motion correction, which adjusts for small fetal and maternal movements occurring during the several second intervals required for magnetization recovery between the acquisition of individual T2 preparation images, that the straightforward determination of T2 from the T2 map becomes feasible. Without motion correction, accurate T2 values could presumably still be obtained by placing individual ROIs over the vessel lumen on each individual T2 preparation image, although this would be more time consuming. One aspect of using the imaging sequence devised by Giri et al. is that the magnetization recovery intervals and triggers for acquiring the readout are based on an ECG signal. For fetal imaging, it is therefore necessary to produce an artificial ECG signal to run the acquisition. Most modern MRI systems have the facility to produce such a signal, and the R-R interval can be programmed according to the estimated fetal heart rate, a process we have termed pseudo-gating. We generally measure the fetal heart rate with a cardiotocograph for several minutes prior to MRI in order to determine the average fetal heart rate for each case and use this for the sequences requiring pseudo-gating. A second longer R-R interval is chosen for the sequences using MOG, to ensure that the k-space data used to reconstruct these images is oversampled.
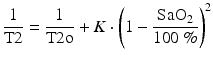
Once the T2 of each vessel of interest is measured, a conversion to SaO2 can be undertaken. We are currently attempting to calibrate in vitro measurements of T2 to the SaO2 of human umbilical cord blood, in order to provide accurate human fetal MR oximetry. In the meantime, we have used the sequence developed by Giri et al. with the parameters shown in Table 10.1 to measure T2 in adult blood samples with a hematocrit matching late gestation fetal blood in order to convert T2 to SaO2. The samples were prepared by exposure to nitrogen gas to have a range of SaO2 to simulate blood in various fetal vessels. The calibration curve and a term fitting T2 to SaO2 are shown in Fig. 10.2. This conversion assumes that the magnetic properties of fetal and adult hemoglobin are the same, an assumption that is likely to be flawed, and until a calibration using fetal blood is obtained, it may be more appropriate to report MR oximetry in terms of vessel T2 rather than SaO2.
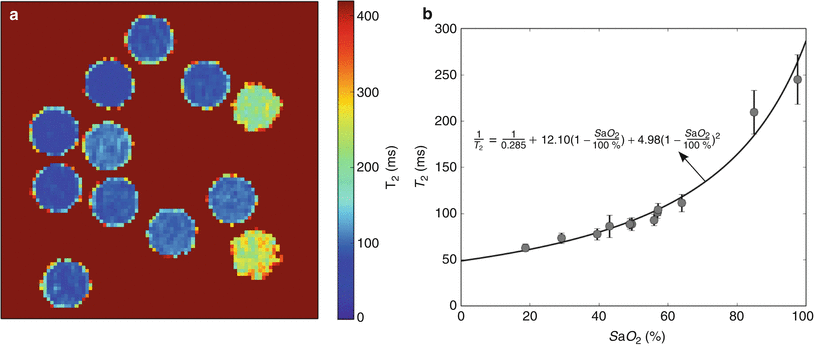
Table 10.1
Proposed sequence parameters for performing cardiovascular magnetic resonance in the late gestation fetus
Sequence | Type | Gating | Resp. comp. | Parallel imaging factor | NSA | TE (ms) | TR (ms) | Slice thick (mm) | Matrix size | FOV (mm) | Temp. resol. (ms) | Scan time (s) |
---|---|---|---|---|---|---|---|---|---|---|---|---|
3D-SSFP | 3D | – | Breath-hold | 2 | 1 | 1.74 | 3.99 | 2 | 256 × 205 × 80 | 400 | – | 13 |
Static SSFP | 2D | – | – | – | 1 | 1.3 | 6.33 | 4 | 320 × 211 | 350 | 1336 | 24 (15 slices) |
Cine SSFP | 2D | MOG | – | 2 | 1 | 1.26 | 3.04 | 5 | 340 × 310 | 340 | 46 | 55 (10 slices) |
Phase contrasta | 2D | MOG | – | – | 1 | 3.15 | 6.78 | 3 | 240 × 240 | 240 | 54 | 36 |
T2 mappingb | 2D | PG | – | 2 | 1 | 1.15c | 3.97c | 6 | 224 × 181 | 350 | 4000 | 12 |
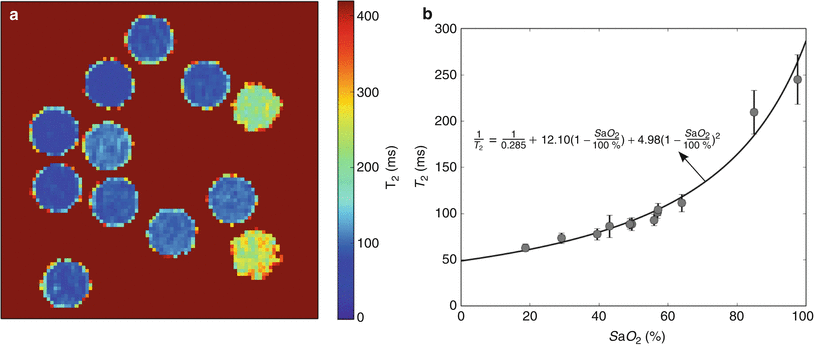
Fig. 10.2
Calibration of the relationship between T2 and SaO2. (a) T2 map of in vitro blood samples with (b) the corresponding calibration curve showing the relationship between T2 and SaO2. The T2 map in (a) was acquired with a T2 preparation sequence with SSFP readout and constructed from 5 images with a range of T2 preparation times spaced evenly between 0 and 200 ms, using a simulated ECG signal for triggering at the estimated fetal heart rate: TE 1.15 ms, TR 3.97 ms, parallel imaging factor 2, slice thickness 5 mm, matrix size 224 × 181, FOV 350 mm, interval between images 4 s, and scan time 16 s. Data points and error bars in (b) represent the mean and standard derivation obtained from a manually drawn region of interest (ROI) within each vial on the T2 map. The solid line represents the fitted relationship between T2 and SaO2. The equation for this relationship, which was used to calculate SaO2 values from fetal T2 measurements, is shown on the plot
10.4 MR Measurement of Fetal Hemoglobin Concentration
A potential source of error in the oximetry method described above is the requirement for an estimation of fetal hemoglobin concentration. While reference data indicates a fairly narrow range of hemoglobin concentration is present in utero [32], we are currently lacking sufficient data for reliable reference ranges in late gestation fetuses. Furthermore, fetal anemia resulting from allo-immunization and viral infection is not uncommon, and chronic hypoxia induces fetal polycythemia [42]. We recognize therefore that the use of an estimated fetal hemoglobin concentration represents a potentially important source of error in fetal MR oximetry based purely on the quantification of T2. However, there may be an elegant solution to this problem that takes advantage of the strong relationship that exists between the T1 relaxation of blood and its hematocrit with higher hematocrits resulting in shorter T1s [15]. Therefore, while the T2 of blood is primarily affected by SaO2, but weakly impacted by hematocrit, its T1 is primarily influenced by hematocrit, but weakly affected by SaO2. We have recently shown that these two relationships can be rearranged in a cubic polynomial equation whereby, with the exception of samples with very high SaO2, there is a single solution for SaO2 and hematocrit for any pair of T1 and T2 values that might coexist in an individual blood sample [38]. A computer simulation showing contour lines for oxygen saturation and hematocrit based on this solution is shown in Fig. 10.3. This work includes a validation of the technique, whereby good agreement is shown between conventional blood gas analysis of adult blood samples manipulated to have a range of oxygen saturations and hematocrits with values predicted for those samples based on their T1 and T2 values measured by MRI in vitro (Fig. 10.4). We anticipate further calibration using umbilical cord blood will confirm that the combination of T1 and T2 mapping will then form the basis of an accurate noninvasive technique for determining the oxygen content of fetal blood in vivo.
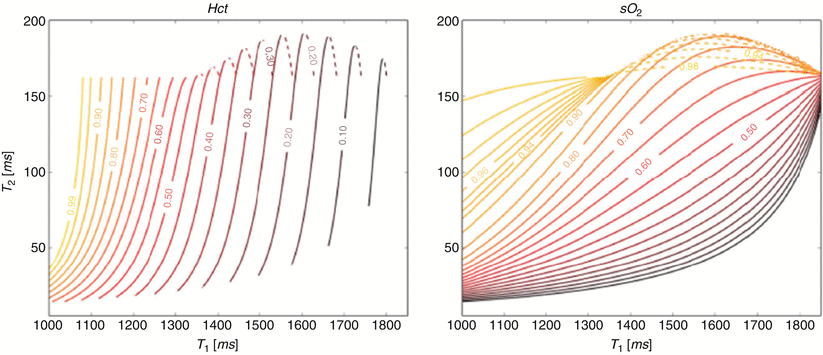
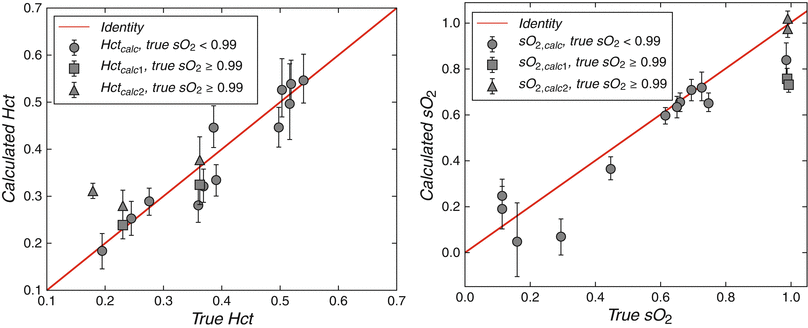
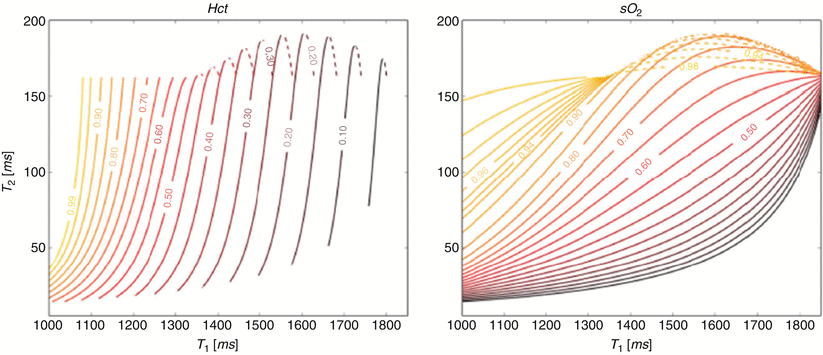
Fig. 10.3
Contour plots of hematocrit (T1,T2) and SaO2 (T1,T2). Note the region of overlap between two solutions (as indicated by solid and dashed contour lines) in the right upper quadrant
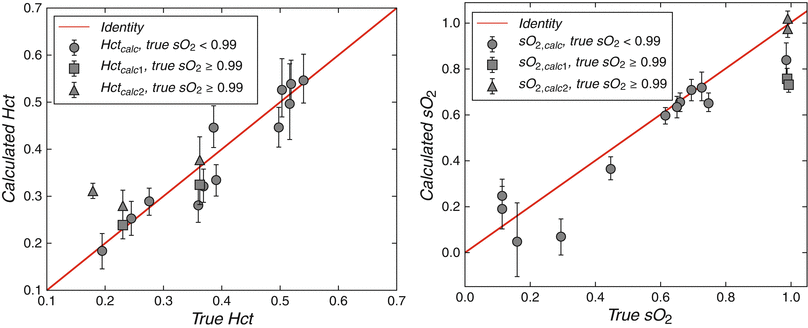
Fig. 10.4
Correspondence between true values of the hematocrit (HCt) and oxygen saturation (SaO2) and those obtained using in vitro T1 and T2 of adult blood indicating good agreement as the basis for a non-invasive technique for measuring the oxygen content of fetal blood in utero
10.5 Measurement of Blood Flow Across the Fetal Circulation Using Phase-Contrast MRI
While fetal color and pulsed Doppler techniques provide a wealth of hemodynamic information about the fetal circulation and have been used to calculate combined ventricular output (CVO) and umbilical blood flow [19, 29, 50, 55], ultrasound measurements of vessel flow are prone to inaccuracy [11]. Although inferior spatial resolution and motion artifact degrade flow measurements made with cine PC CMR and currently limit the technique to late gestation fetuses, this approach avoids some of the difficulties encountered with ultrasound flow measurements. These include problems with obtaining an adequate angle of insonation and inaccuracies resulting from difficulties in measuring vessel area and with accounting for the faster velocities of blood flow in the center of the vessel and slower flow adjacent to the vessel wall. Under ideal conditions, PC CMR is more accurate than ultrasound for blood flow quantification, and criteria for acquiring adequate temporal and spatial resolution to achieve accurate flow measurements in small vessels have been reported [17, 24]. We attempted to assess the accuracy of PC CMR with MOG using flow phantoms and by comparing measurements made in the neck vessels of exercising adult volunteers using conventionally gated PC CMR measurements with those made using MOG [18, 48]. The results of this comparison are shown in Fig. 10.5 and indicate that flow measurements made in the larger fetal vessels of a human fetus close to term are likely to be accurate. Further reassurance regarding the accuracy of fetal PC MRI is to be found in the good correlation between direct and indirect measurements of pulmonary blood flow made using PC CMR [40]. Figure 10.6 shows good agreement between pulmonary blood flows calculated as the difference between main pulmonary artery and ductus arteriosus flow versus the sum of the right and left pulmonary arteries in normal late gestation human fetuses.
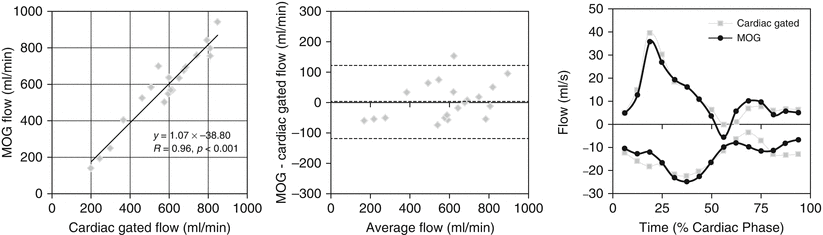
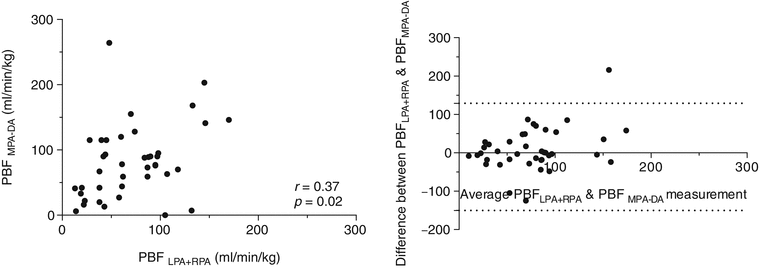
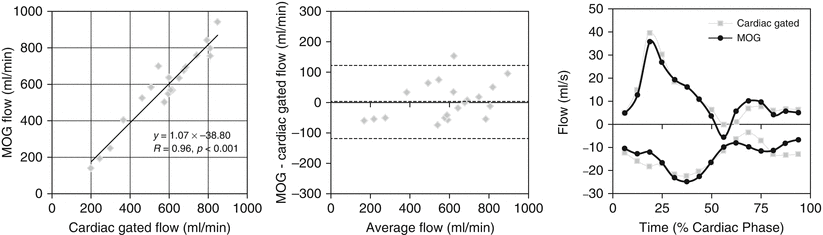
Fig. 10.5
Validation study of five adult volunteers comparing conventional vs MOG measurement of flow. Measurements from the common carotid arteries and jugular veins made using conventional cardiac-gated PC CMR compared with the same measurements made by PC CMR with MOG. The scatterplot shows agreement between the two methods, while the Bland-Altman plot reveals negligible bias. Flow curves measured in the right common carotid and right internal jugular vein by the two methods for one volunteer are shown
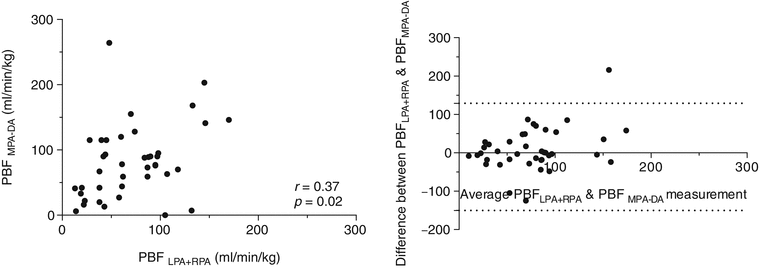
Fig. 10.6
Internal validation of flow measurements for all 40 fetuses comparing pulmonary blood flow measured directly as the sum of right and left pulmonary arterial flows versus an indirect measurement: the difference between main pulmonary artery and ductus arteriosus flows showing reasonable agreement between the two measures with no significant bias (bias: 10 ml/min/kg, SD of bias 57.3, 95 % limits of agreement from −102.4 to 122.4 ml/min/kg)
One approach to measuring the distribution of the fetal circulation is to use PC CMR flow measurements made in the ascending aorta (AAo), main pulmonary artery (MPA), ductus arteriosus (DA), right and left branch pulmonary arteries (RPA & LPA), superior vena cava (SVC), descending aorta (DAo), and umbilical vein (UV). The CVO can be derived from the sum of AAo and MPA flows, although we have always added 3 % to this figure to account for coronary blood flow, based on the reports of previous fetal lamb experiments [44]. Foramen ovale (FO) flow can be estimated as the difference between the left ventricular output and pulmonary blood flow, as FO flow and pulmonary blood flow are the two alternative sources of filling of the left heart. In order to measure umbilical flow, we target the UV in the liver, several centimeters beyond the umbilicus, but also well proximal to the porta hepatis. The UV takes a relatively straight course in its intrahepatic segment, and this permits PC CMR measurements that avoid the more complex flow patterns that occur in its free loops. Our approach has been to copy the PC CMR slice prescriptions to perform consecutive T2 maps in each of the larger branches (AAo, MPA, DA, SVC, DAo, UV) [46]. One approach to obtaining these flow measurements is shown in Fig. 10.7, and the imaging parameters for each of the sequences we use are shown in Table 10.1. An important principle to follow when acquiring the flow and oximetry measurements is to use two perpendicular long-axis views of each vessel in order to prescribe the slice position. This will ensure the imaging plane is acquired in a true short-axis orientation to the vessel, thus minimizing the chance of partial volume artifacts in the resulting images. We acquire surveys of the fetal thorax in three orthogonal planes using stacks of static SSFP slices with the sequence parameters shown in Table 10.1 in order to guide the subsequent PC and T2 prescriptions. These surveys may need to be repeated several times during the study to relocate the vessels accurately following fetal or maternal movement.
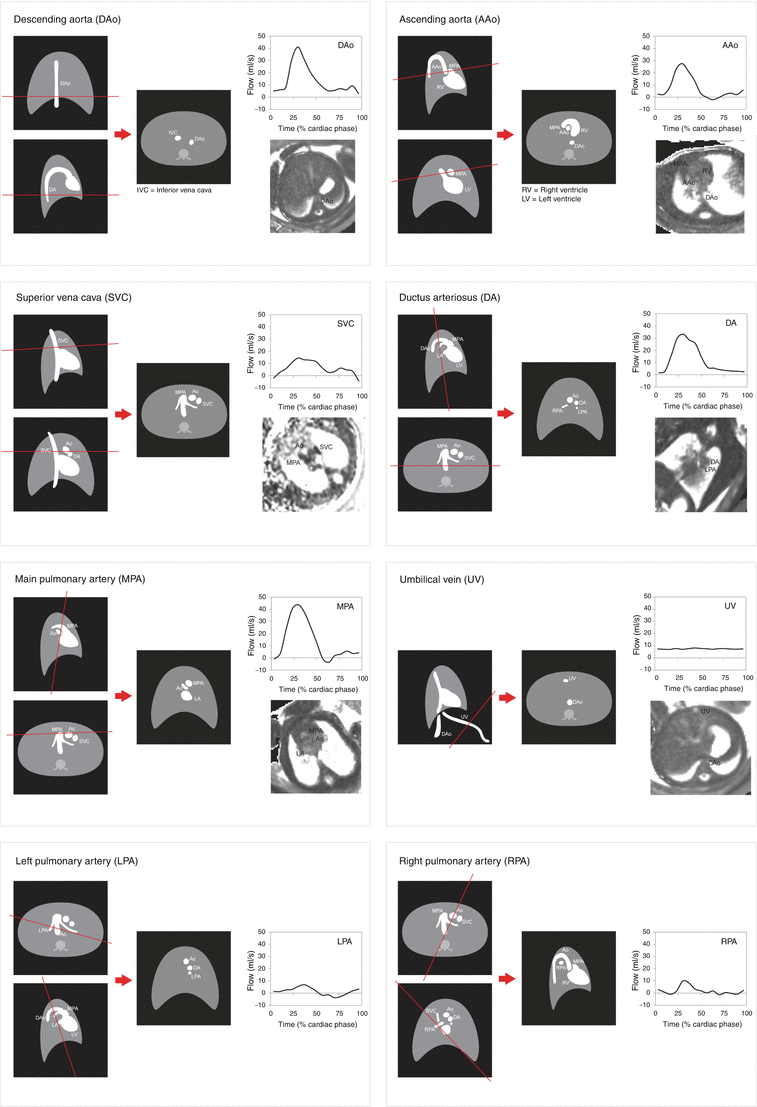
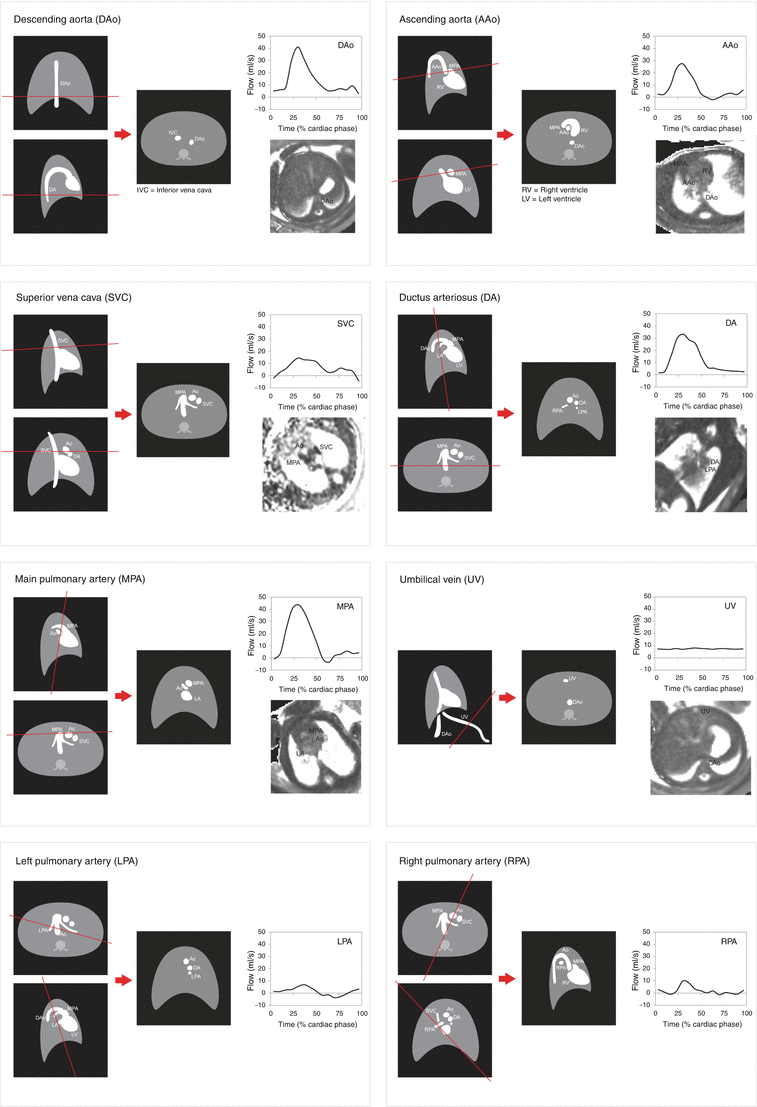
Fig. 10.7
Orientation of phase-contrast (PC) and T2 acquisitions from coronal, sagittal, and axial localizers showing expected appearances of modulus PC images, representative flow curves and examples of T2 maps for each of the target vessels
Metric optimization of the PC CMR measurements is currently performed using a purpose designed MATLAB program, which is available as an open-source internet resource [27]. Following MOG, the individual PC files are transferred back to the magnet computer, which converts them to DICOM files that can be processed using conventional CMR software for flow quantification. We use Q-Flow (Medis, Netherlands) to place regions of interest around the target vessel in order to determine vessel flow in ml/min. Vessel flows can be indexed to fetal weight by measuring fetal volume. One such approach involves the acquisition of a three-dimensional SSFP acquisition of the whole fetus. The stark contrast between the high signal of amniotic fluid and dark signal of the fetal skin on this sequence makes automatic segmentation of the fetal envelope reasonably straightforward using a threshold approach. We use Mimics by Materialize (Leuven) for this and have traditionally applied the following previously reported conversion of fetal volume to fetal weight [3]:
![$$ \mathrm{Fetal}\;\mathrm{weight}\;\left(\mathrm{g}\right)=\left[\mathrm{fetal}\;\mathrm{volume}\;\left(\mathrm{ml}\right)+120\right]\times 1.03 $$](/wp-content/uploads/2016/10/A330004_1_En_10_Chapter_Equb.gif)
![$$ \mathrm{Fetal}\;\mathrm{weight}\;\left(\mathrm{g}\right)=\left[\mathrm{fetal}\;\mathrm{volume}\;\left(\mathrm{ml}\right)+120\right]\times 1.03 $$](/wp-content/uploads/2016/10/A330004_1_En_10_Chapter_Equb.gif)
10.6 Calculation of Fetal Oxygen Delivery and Consumption
The combination of vessel flow and oximetry that is made possible using PC CMR and MR oximetry can be used to quantify placental function and fetal metabolism by allowing the measurement of fetal oxygen delivery (DO2) and consumption (VO2). Fetal DO2 is the product of UV flow and UV oxygen content. The oxygen bound to hemoglobin and oxygen dissolved in plasma comprises the oxygen content of blood. In adult blood, the contribution to blood oxygen content from oxygen dissolved in plasma is in the 2–3 % range, while in fetal blood, this proportion is even lower, so that fetal blood oxygen content can be assumed to be the amount of oxygen combined with hemoglobin [44]. For adult blood, the amount of oxygen bound to hemoglobin can be calculated from the oxygen saturation (SaO2) and hemoglobin concentration [Hb]:
where 1.36 is the amount of oxygen in ml that can be bound to 1 g of hemoglobin at 1 atm [44]. This figure is likely to be similar for fetal blood, as the total oxygen-carrying capacity of fetal hemoglobin is the same as adult hemoglobin despite the higher affinity of fetal hemoglobin for oxygen.
![$$ \mathrm{Oxygen}\;\mathrm{content}\;\left(\mathrm{ml}\right)={\mathrm{SaO}}_2\times \left[\mathrm{H}\mathrm{b}\right]\times 1.36 $$](/wp-content/uploads/2016/10/A330004_1_En_10_Chapter_Equc.gif)
Fetal VO2 is given by the product of UV flow (Q UV) and the difference between the oxygen contents (ΔC) of the UV and the umbilical artery (UA):
Because of the small size of the UA, the T2 in the descending aorta (DAo) is used for this calculation.

If it is assumed that the majority of the flow in the superior vena cava (SVC) is venous return from the brain, then fetal cerebral VO2 can also be approximated:


10.7 Further Technical Considerations
10.7.1 Field Strength
Fetal CMR can be performed on 1.5 and 3 T systems. 1.5 T systems are less prone to SSFP banding artifacts resulting from magnetic field inhomogeneity. However, the increased SNR available at 3 T makes PC CMR more robust and facilitates MOG reconstruction. An important consideration is the effect of field strength on the relationship between T2 and SaO2, with shorter T2 values encountered at 3 T [22].
10.7.2 Patient Positioning and Coil Selection
A body-matrix coil placed on the maternal abdomen, as close to the fetal thorax as possible, combined with a spine coil provide the best signal for fetal imaging. The addition of a second surface coil placed around the mother’s back may help to improve signal across the whole field of view, if the mother is in a lateral decubitus position, which many women find most comfortable in later on in pregnancy.
10.8 The Normal Fetal Circulation
10.8.1 Existing Data
The interpretation of fetal flow and oximetry measurements requires an understanding of normal fetal cardiovascular physiology. Many of our concepts about the normal fetal circulation are derived from extensive experiments performed using invasive techniques in the ovine fetus. Abraham Rudolph and his collaborators used radioactive microspheres injected into different venous compartments in conjunction with conventional blood gas analysis from various arteries and veins to define the distribution of flow and blood oxygen content across the fetal circulation [44]. Further information about the distribution of flow in the human fetal circulation has been obtained by a number of groups using ultrasound [19, 20, 29, 50, 55]. These confirm that in common with the lamb fetus, the human fetal circulation operates in parallel with shunts at the foramen ovale and ductus arteriosus resulting in blood bypassing the fetal lungs. This is tolerated in the fetal circulation because gaseous exchange occurs at the placenta. The fetus exists in a relatively low-oxygen environment but also has lower VO2 than the newborn due to lower demands for thermoregulation [44].
In fetal lambs, the SaO2 of blood in the left side of the fetal heart is approximately 10 % higher than the right due to a remarkable streaming mechanism where oxygenated blood returning from the placenta is preferentially directed across the foramen ovale via the left liver and ductus venosus [44]. This mechanism presumably exists to ensure a reliable source of oxygen to the developing brain and pumping heart. The less well-oxygenated blood returning from the SVC and lower body is preferentially routed towards the tricuspid valve and then onto the ductus arteriosus and pulmonary circulation. As pulmonary vascular resistance in the third trimester is inversely proportional to the oxygen content of the blood in the pulmonary arteries, a high pulmonary vascular resistance is maintained in the fetal lamb [44].
10.8.2 Distribution of Blood Flow
Based on a combination of measurements in fetal lambs and consideration of the greater size of the human brain, as well as ultrasound measurements of pulmonary blood flow in the human, Rudolph predicted the distribution of blood flow and oxygen saturations across the human fetal circulation [44]. A comparison of those estimates with our findings in 40 normal late gestation human fetuses is shown in Table 10.2. Reference ranges for the distribution of the normal late gestation human fetal circulation are shown in Table 10.3. The results of the MRI findings in this preliminary cohort are also shown in Figs. 10.8 and 10.9. The MRI findings are remarkably similar to Rudolph’s estimates, with a mean CVO of 465 ml/min/kg, and suggest that as in lambs, the human right ventricle provides a slightly larger contribution to the CVO than the left. Two thirds of the right ventricular output passes across the DA, with the remainder passing into the pulmonary circulation. Pulmonary blood flow therefore accounts for about 15 % of the CVO or 75 ml/min/kg. About ¾ of the left ventricular output passes into the head and upper limbs and drains back to the SVC, which carries about 135 ml/kg/min, which is about 30 % of the CVO. The remaining 10 % of the CVO passes around the aortic isthmus, joining the ductus arteriosus flow to provide about 250 ml/min/kg, a little over half of the CVO to the DAo. According to our MRI measurements, a little over half of this DAo flow is directed back to the placenta via the umbilical circulation. This measurement is lower than Rudolph’s estimates but more in keeping with a number of subsequent ultrasound studies. One possible explanation for the finding of lower umbilical flow in the human compared with the lamb is the higher oxygen-carrying capacity of human fetal blood compared with the human resulting from the higher concentration of hemoglobin in human fetal blood [44]. This higher oxygen-carrying capacity would allow the placenta to deliver the same amount of oxygen to the fetus despite the lower umbilical flow.
Table 10.2
Comparison of the distribution of the fetal circulation in the late gestation human measured by phase-contrast MRI and the late gestation fetal lamb measured using radioactive microspheres [44]
CVO | MPA | AAO | SVC | DA | PBF | DAO | UV | FO | ||
---|---|---|---|---|---|---|---|---|---|---|
Mean flows (ml/min/kg) | Human MRI | 465 | 261 | 191 | 137 | 187 | 74 | 252 | 134 | 135 |
Lambs | 450 | 250 | 185 | 140 | 175 | 75 | 220 | 180 | 125 | |
Mean flows (% of CVO) | Human MRI | 56 | 41 | 28 | 41 | 15 | 54 | 29 | 29 | |
Lambs | 56 | 41 | 31 | 39 | 17 | 49 | 39 | 28 |
Table 10.3
Means, standard deviations, and reference ranges (mean ± 2SD) of flows in 40 normal late gestation human fetuses measured by phase-contrast MRI expressed in ml/min/kg and as percentages and as modeled mean percentages of the combined ventricular output
CVO | MPA | AAO | SVC | DA | PBF | DAO | UV | FO | |
---|---|---|---|---|---|---|---|---|---|
Mean flow (ml/min/kg) | 465 | 261 | 191 | 137 | 187 | 74 | 252 | 134 | 135 |
SD | 57 | 46 | 35 | 30 | 39 | 43 | 46 | 36 | 49 |
Mean ± 2 SD | (351,579) | (169,353) | (121,261) | (77,197) | (109,265) | (0,160) | (160,344) | (62,206) | (37,233) |
Mean flow (% CVO) | 56 | 41 | 29 | 40 | 16 | 55 | 29 | 29 | |
SD | 6 | 6 | 7 | 8 | 9 | 10 | 9 | 11 | |
Mean ± 2 SD | (44,68) | (29,53) | (15,43) | (25,57) | (0,34) | (35,75) | (11,47) | (7,51)
![]() Stay updated, free articles. Join our Telegram channel![]() Full access? Get Clinical Tree![]() ![]() ![]() |