Fig. 3.1
Coronal (a) and sagittal (b) SSFSE of the gravid uterus characterized by oligohydramnios. Images demonstrate reasonable resolution of the fetal brain despite the lack of amniotic fluid, which is a significant limitation for fetal ultrasound
Fetal MRI should be considered a study performed with the precise aim of clarifying a diagnostic doubt or query, not as a screening tool [11]. A sonographic anatomic survey should address the clinical questions via a tailored examination. Moreover, review of a fetal MRI should be performed in conjunction with the level II US with fetal MRI considered a level III technique.
3.2.2 MRI System
Current recommendations are for the use of a 1.5-T field strength for fetal imaging. An adequate signal-to-noise ratio (SNR) will be obtained at this field strength [11].
Lower field strengths will suffer from image noise, whereas, higher field strengths are still being validated. The coils chosen for fetal MRI can vary based on maternal size or fetal gestational age. Multichannel phased array or cardiac surface coils should grant the best signal, and the coils should be as close to the region of interest as possible. Spine coils may be needed to allow a greater field of view later in pregnancy.
Each MRI vendor will have proprietary names for specific sequences that are useful in fetal MRI. Commonly used sequences with vendor names are listed in Table 3.1.
Table 3.1
Commonly used MRI sequences used in fetal magnetic resonance imaging by vendor
GE | Siemens | Phillips | Toshiba | Hitachi | |
---|---|---|---|---|---|
Sequence type | |||||
Spin echo | SE | SE | SE | SE | SE |
Gradient echo | GRE | GRE | Fast field echo | GE | Field echo |
Spoiled Gradient echo | SPGR | FLASH | T1 FFE | Fast FE | SARGE |
Coherent Gradient echo | GRASS | FISP | FFE | Rephased SARGE | SSFP |
Stead-state Free precession | SSFP | PSIF | T2 FFe | Time-reverse SARGE | |
TrueFISP | FIESTA | TrueFISP | Balanced FFE | Balanced SARGE | True SSFP |
TrueFISP/dual excitation | FIESTA-C | CISS | Phase-balanced SARGE | ||
Multi-echo data image Combination | MERGE | MEDIC | M-FFE | ||
Ultrafast gradient echo | Fast SPGR | TurboFLASH | TFE | Fast FE | RGE |
Ultrafast gradient echo 3D | 3D fast SPGR | MPRAGE | 3D TFE | 3D fast FE | MPRAGE |
Volume-interpolated GRE | LAVA-XV | VIBE | THRIVE | TIGRE | |
Body diffusion | REVEAL | DWIBS | Body Vision | ||
Susceptibility-weighted imaging | SWAN | SWI | (Venous BOLD) | ||
Inversion recovery | IR, MPRI, FastIR | IR, Turbo IR | IR-TSE | IR | IR |
Short tau IR | STIR | STIR | STIR | Fast STIR | STIR |
Long tau IR | FLAIR | Turbo Dark Fluid | FLAIR | Fast FLAIR | FLAIR |
Turbo spin echo/fast spin Echo | FSE | TSE | TSE | FSE | FSE |
Single-shot TSE/FSE | SSFSE | HASTE | SSTSE | FASE | SSFSE |
3D TSE with variable flip angle | CUBE | SPACE | VISTA | ||
Echoplanar imaging | EPI | EPI | EPI | EPI | EPI |
Diffusion-weighted imaging | DWI | DWI | DWI | DWI | DWI |
Apparent diffusion coefficient map | ADC | ADC | ADC | ADC | ADC map |
Turbo gradient spin echo | TurboGSE | GRASE | Hybrid EPI |
3.2.3 Protocol
The basic sequences for performing fetal MRI are available on any vendor platform. Table 3.2 is a typical starting protocol that we use at our institution. The number of series obtained for any given sequence will vary on a case-by-case basis, as fetal motion often necessitates repeating sequences until the images are adequate. Moreover, fetal MRI protocols are often adapted during the examination depending on the clinical question. Most studies are performed in the early morning, following an overnight fast or a fasting period of at least 4–6 h, to limit postprandial motion. In addition to maternal fasting, most institutions stop maternal ingestion of prenatal vitamins for at least 1 day prior to the study, since prenatal vitamins are high in iron, which may cause localized field inhomogeneities during the time of the study. Finally, the mother should empty her bladder just prior to the start of imaging.
Table 3.2
Basic fetal MRI protocol used at our institution (protocol based on a 1.5-T GE magnet)
FOV (MM) | Thickness/gap (mm) | Matrix | TR (ms) | TE (ms) | Flip angle (degrees) | Number of slices | Time (s) | NSA | |
---|---|---|---|---|---|---|---|---|---|
3-plane SSFSE survey | 480 | 10/10 | 256/179 | 1200 | 94 | 150 | 24 | 20 | 1 |
Fetal SSFSE neural and body | 360 | 3–4/0–4 | 256/179 | 1200 | 94–120 | 150 | 22 | 20 | 1 |
FIESTA | 280 | 3.5/0 | 256/230 | 3.97 | 1.99 | 70 | 18 | 19 | 2 |
Fetal SSFSE neural and body | 260 | 3/0 | 256/179 | 1200 | 90 | 150 | 45 | 56 | 1 |
Fetal neural DWI/ADC | 230 | 5/0 | 192/100 | 6300 | 89 | 90 | 20 | 96 | 3 |
Fetal neural and body SPGR ± fat suppression | 280 | 4–5/0.5 | 256/192 | 175 | 2.84 | 70 | 20 | 20 | 1 |
Appropriate patient positioning cannot be overstated. The mother needs to be in a position of comfort so as to limit any maternal motion. Typically, the patient is placed supine or in a left lateral position. Supine positioning may be uncomfortable in late pregnancy, as the size of the uterus may cause vena cava compression or compression of maternal organs.
Initial imaging is performed with a fast localizer sequence, typically a large field ultrafast T2-weighted single-shot sequence, in the maternal coronal, axial, and sagittal planes. These images are used to determine the placental and fetal positioning with respect to the mother. The fetus is more often than not positioned in an oblique axis to the standard maternal axis. Once fetal lie is determined, the images obtained from the localizer sequences are used as scout images for the subsequent sequences. Each additional series also serves as a scout for localization to adjust for fetal repositioning.
Just as plane selection must be made for the fetus relative to scout images of the maternal pelvis, so must plane selection of the fetus be adjusted for each fetal body region. In other words, true anatomic planes can vary by body region when the fetus is curled (Fig. 3.2). For example, the axial plane of the calvarium typically is not in the same axial plane as the fetal chest and abdomen. Thus, constant attention to image acquisition by the technologist is paramount.
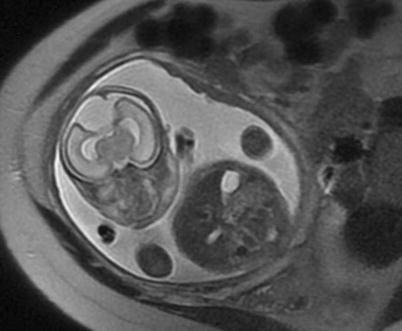
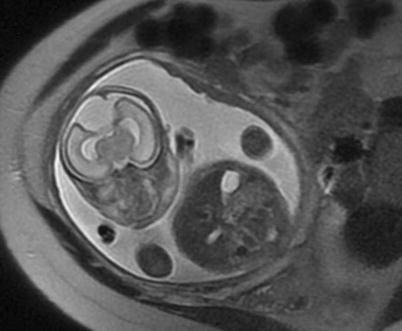
Fig. 3.2
Axial SSFSE image through the maternal pelvis shows the variability of fetal positioning. This image provides a near-coronal image through the fetal brain and an axial image of the fetal abdomen. Attention to acquisition planes is critical to confirm landmarks and normal organ configurations
To obtain high-resolution images, the field of view should be as small as possible without causing aliasing artifact. Slice thickness may be adjusted as needed to image the system or organ of interest, with an optimal range of 3–4 mm to achieve high SNR and through-plane resolution [12, 13]. Decreasing matrix size is another parameter influencing SNR, with smaller matrix sizes providing increased signal.
The trade-off with smaller matrix sizes is decreased in-plane resolution. Breath holding can reduce motion-related artifact, although the ultrafast sequences do allow for free-breathing acquisition [13]. Parallel imaging can be used to improve temporal resolution as long as care is exercised with selecting the field of view and with addressing SNR challenges [14]. All parameters should be chosen to keep scan time to a minimum.
3.3 Sequences and Artifacts
3.3.1 T2-Weighted Imaging
The ultrafast T2-weighted sequence is the recognized workhorse in fetal MRI [13], allowing excellent visualization of the fetal anatomy, especially cerebral anatomy. T2-weighted imaging allows optimization of tissue contrast in the fetus, due to the large volume of water in the fetus and adjacent amniotic cavity. Anatomic detail of the fetal brain, fluid-filled cavities, lungs, placenta, and fetal profile is consistently strong [15]. SSFSE allows image acquisition during a single TR interval with a total acquisition time of less than 2 s per image [16]. Images should be acquired with a 1–2 s time delay between each image to prevent saturation effects that will degrade the signal-to-noise ratio (SNR). Alternatively, the slices can be acquired in an interleaved fashion with a gap equal to the slice thickness to minimize the potential signal loss from bulk-fluid motion artifacts and cross talk. In neuroimaging, longer TEs allow better discrimination of the grey-white matter interface in the fetus since the T2 contrast increases with increasing TE times [17].
3.3.2 Steady-State Free Precession
Fast imaging with balanced steady-state free precession (SSFP) provides images with T2/T1 contrast weighting with high temporal resolution [18–21]. These sequences are especially useful in demonstrating vasculature and fluid-filled cavities, specifically those surrounded by dense tissues, and these sequences can be used to visualize the fetal heart chambers [15]. Evaluation of the umbilical cord and its insertion may also be enhanced with this sequence.
SSFP sequences are obtained in a wide field of view, allowing visualization of the maternal abdominal anatomy and the uterus/placenta. Large field of view imaging limits usefulness of SSFP sequences in fetal brain imaging in early gestation [15].
SSFP provides similar image quality to SSFSE for brain imaging in the second trimester; however, axonal migration in the third trimester is best depicted with SSFSE [19]. SSFP is inferior to SSFSE in assessing the fetal lungs prior to 25 weeks gestational age since the lungs demonstrate hyperintense signal only in advanced gestation.
3.3.3 T1-Weighted Imaging
T1-weighted images are acquired using a gradient-echo sequence with breath-hold fast spoiled gradient-echo sequences used most commonly [15]. T1-weighted sequences provide little information over SSFSE sequences but can increase sensitivity for detection of fat, calcification, hemorrhage, or proteinaceous structures. Fat-suppressed T1-weighted images increase the dynamic range of fetal MRI and allow more specific detection of fat and hemorrhage [22].
T1-weighted imaging has proven beneficial when evaluating certain normal structures as well as a few common pathologies. The pituitary and thyroid glands can be seen at 20 weeks [15]. The liver is distinguished by its hyperintense T1 signal due to its high iron affinity, a fact exploited in evaluation for congenital diaphragmatic hernias or abdominal wall defects with displaced liver [23, 24].
3.3.4 DWI/DTI
Diffusion imaging is challenging in fetal MRI. Nevertheless, one important use is its sensitivity for detecting ischemic lesions, brain lesions, and regions of placental infarction [27, 28]. Certain diffusion sequences have been used to visualize the maturational processes of the cerebral cortex and premyelinating white matter, but this technique in fetal MRI is still in research [29–32]. Diffusion anisotropy of the white matter tracts increases constantly from premyelinating to myelinating stages, revealing maturing white matter tracts before there are visualized changes on T1- and T2-weighted images [13, 32].
3.3.5 Advanced Imaging Techniques
Spectroscopy in fetal MRI has been applied to the older fetus whose head is relatively nonmobile within the maternal pelvis [33, 34]. Inversion recovery sequences may be used to obtain additional information involving the fetal brain, but these are sensitive to hemorrhage [15]. Dynamic fetal imaging allows assessment of fetal swallowing, palatal defects, diaphragm motion, peristalsis, and gross fetal motion [35].
Finally, echoplanar imaging may be considered on occasion. The speed at which echoplanar imaging (EPI) is acquired allows it to overcome typical motion artifacts seen in conventional MRI [36]. EPI has been used to produce ungated fetal cardiac movies, for volumetric measurements and for evaluating hepatic hematopoiesis [37–39]. EPI is unique in depicting the fetal skeleton and cartilaginous epiphysis in the fetus before 27 weeks gestation [15]. The magnetic susceptibility of EPI makes it valuable in detecting hemosiderin and certain placental pathologies [15].
The use of 3 T field strength to perform fetal MRI is currently under investigation. The most important advantage to 3 T imaging would be the gain in SNR, which is especially important in small part imaging [40]. On the other hand, 3 T field strength exaggerates artifacts, such as large field inhomogeneity, magnetic susceptibility, and chemical shift artifacts [40]. Lastly, fetal safety at 3 T, including static field exposure, gradient field switching, and radiofrequency power deposition, is a topic of research [40].
3.3.6 Gadolinium-Based Contrast Agents
Routine use of gadolinium-based contrast agents is not a standard of care, since their biosafety in pregnancy has not been established [41]. Gadolinium-chelate agents cross the placenta, are filtered by the kidneys, are excreted into the amniotic fluid, and are resorbed by the fetus through the swallowing of amniotic fluid. With the cycle of excretion and reabsorption through swallowing, the biological half-life of gadolinium in the fetus is not known [41]. With the accumulation of gadolinium-chelate agents in the amniotic fluid, there is potential for dissociation of the toxic-free gadolinium ion, which poses a risk for the development of nephrogenic systemic fibrosis (NSF) in the mother or child. If gadolinium-based contrast agent is deemed necessary in a pregnant patient, then the agents with the lowest risk for development of NSF should be used at the lowest dose that allows for diagnosis.
3.3.7 Common Artifacts
Fetal MRI struggles with artifacts as seen with MRI in other body regions. Motion artifacts, susceptibility, aliasing, partial volume averaging, and Gibbs artifacts are issues at times. Artifact from bulk fluid motion can complicate SSFSE sequence when excited spins in fluid change position with respect to slice and/or spatial encoding gradients before the signal is acquired (Fig. 3.3). If all of the excited fluid remains stationary from excitation to the time of signal acquisition, then the fluid signal will be uniformly hyperintense. Alternatively, if all or a partial volume of the excited fluid moves out of the imaging plane by the time of image acquisition, then the fluid will become hypointense. As long as the fetus is not moving continuously, only one or two adjacent slices should be degraded during a typical SSFSE acquisition.
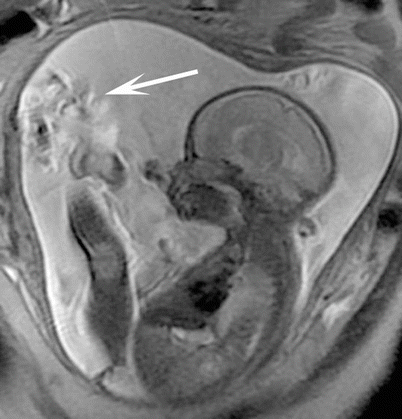
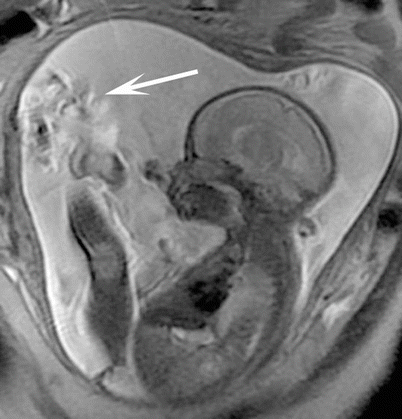
Fig. 3.3
Large field of view SSFSE image of the gravid uterus with sagittal acquisition through the fetus nicely demonstrates linear bands of dark signal (arrow) associated with bulk fluid motion from fetal shifting during image acquisition
3.4 Fetal Anatomy
Interpretation of fetal MRI requires knowledge of normal anatomy during the course of gestation, including size, configuration, position and signal changes in each organ.
The vast majority of questions brought to fetal MRI concern the central nervous system (CNS) and CNS pathology, so issues of cleavage and migration of neuronal structures are as important as gross insults of infarction or hemorrhage. In body imaging, many questions pertain to prognosis and to plans for delivery. We will review an overall approach.
3.4.1 Preparation
Fetal MRI interpretation requires correlation with available US images and/or report. The US provides a road map for tailoring the subsequent MRI examination; valuable time in the scanner should focus on specific clinical questions and on anatomy not cleared by US. Also, the US evaluates structures that are often incompletely imaged during the fetal MRI, such as the extremities or digits.
3.4.2 Situs
Determining fetal situs may not be intuitive. Confirming situs solitus eliminates numerous pathologies and allows lateralization of asymmetric findings. To determine situs, one must orient the fetus relative to the maternal axis (Fig. 3.4). In relation to the maternal long axis, the fetus can thus be vertex (head directed toward the maternal pelvis) or breech (head directed toward maternal head). If the fetus is breech, then the fetal spine is down, and the fetal abdomen is up on the image, making the fetal lie similar to that of the mother. If the fetus is vertex, the fetal spine is down, and the fetal abdomen is up on the image, creating an opposite viewing orientation of fetal and maternal anatomy. Fetal situs can subsequently be determined for any rotation about the fetal axis with regard to breech or vertex positioning.
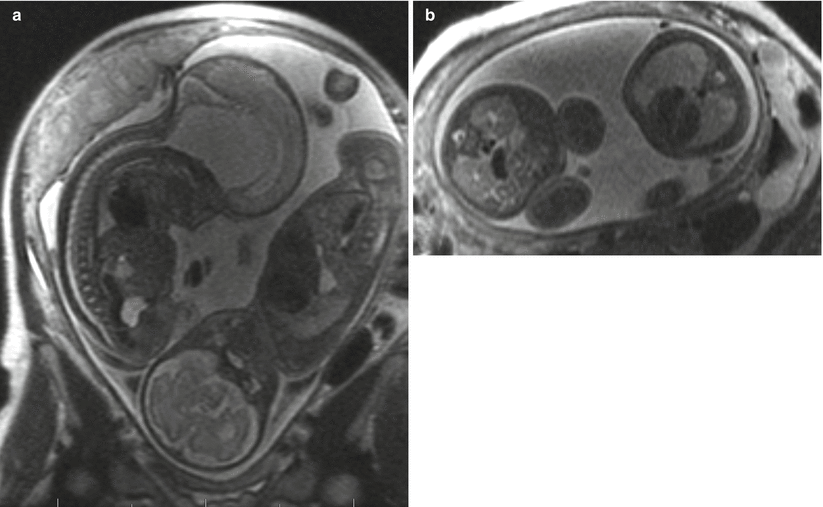
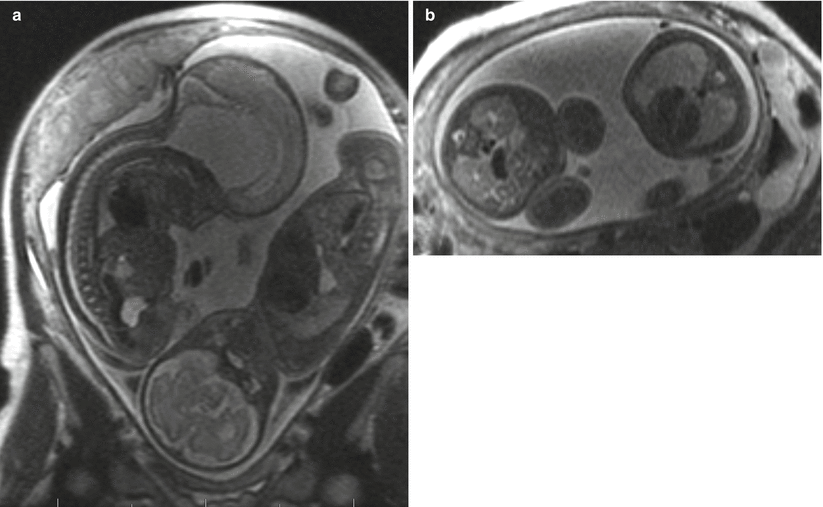
Fig. 3.4
Coronal (a) and axial (b) SSFSE images through the gravid uterus with twin gestation highlight the complexity of determining fetal situs and lateralization of abnormalities. Both fetuses have left side down with respect to the mother, but fetus (a) is vertex and fetus (b) is breech. The most helpful fetal organ for lateralization is the fetal stomach (not shown), except in the case of congenital diaphragmatic hernia.
US can suggest situs ambiguity, but it may not completely describe all the anomalies that can accompany these syndromes. Furthermore, US may miss a situs abnormality, which may inadvertently be discovered on MRI by virtue of the large field of view imaging [42]. Recent work has shown that fetal MRI can demonstrate situs anomalies at least similarly to US as well as associated malformations that provide important information regarding perinatal management [42].
3.4.3 Intracranial Anatomy
The entirety of fetal embryology and neuroanatomy is beyond the scope of this chapter. Dedicated textbooks explore the magnitude of the topic, and several references on brain development are available [43–51]. We will highlight key landmarks and developmental milestones in the fetal brain.
Fetal MRI is commonly used to investigate underlying etiology for ventriculomegaly and morphologic brain abnormalities that are incompletely assessed with US. The brain evolves throughout gestation, making evaluation challenging to those unaware of the normal appearance of the anatomy at a specific gestational age [6].
Beginning in the early second trimester, structures that arise from the telencephalon, mesencephalon, and rhombencephalon become visible with the gyral and sulcal pattern developing in a predictable sequence [52].
The early fetal brain is composed predominately of large ventricles with a thin layer of cerebral tissue having a smooth appearance [6]. The choroid plexus, seen as an echogenic structure filling the lateral ventricles on US, is difficult to visualize early in gestation by MRI [6]. At 14 weeks, the interhemispheric fissure separating the cerebral hemispheres is well developed [6]. At approximately 16 weeks, the Sylvian fissure begins to appear, but the cortex does not undergo infolding and opercular formation before 34 weeks gestation. Normal cortical maturation, while predictable and easily visualized on fetal MRI, often lags behind the maturation described in neuroanatomic specimens [6, 43, 48]. Table 3.3 provides a list of the commonly seen and evaluated sulcal developmental landmarks, their neuropathologic appearance, and the gestational week of detectability at MRI [43, 53]. Note that the neuroanatomic information provided in the tables describes the gestational ages at which 25–50 % of the brains demonstrate a specific cortical landmark [6, 54]. On a midsagittal plane, the cingular sulcus appears at 24 weeks gestation, the marginal at 27 weeks gestation, the parieto-occipital at 22 weeks gestation, and the calcarine at 24 weeks gestation. On a lateral sagittal plane, the central sulcus appears at 27 weeks gestation, the postcentral at 28 weeks gestation, and the precentral sulcus at 27 weeks gestation. On an anterior coronal plane, the superior frontal sulcus and the inferior frontal sulcus appear at the 29th week of gestation. On a coronal plane at the level of the third ventricle, the superior and inferior temporal sulci appear at 27 and 33 weeks, respectively. Sulcation continues throughout fetal life, with secondary sulci becoming evident at 32–35 weeks and tertiary sulci subsequently forming [6] (Fig. 3.5). If CNS imaging is necessary in early pregnancy, recent work is available to assess biometric data below 24 gestational weeks [55].
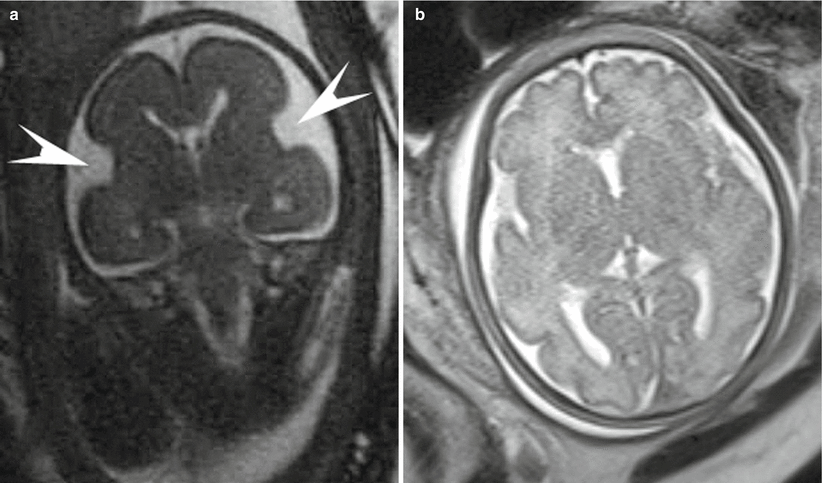
Table 3.3
Fetal sulcation observed on MRIa
Gestational age (week) | ||
---|---|---|
Present in more than 75 % of | ||
Neuropathologic appearanceb | brains in present MR study | |
Sulci of the vertex | ||
Central sulcus | 20 | 27 |
Precentral sulcus | 24 | 27 |
Postcentral sulcus | 25 | 28 |
Sulci of the medial cerebral surface | ||
Interhemispheric fissure | 10 | 22–23 |
Callosal sulcus | 14 | 22–23 |
Parieto-occipital fissure | 16 | 22–23 |
Cingular sulcus | 18 | 24–25 |
Secondary singular sulci | 32 | 33 |
Marginal sulcus | 27 | |
Calcarine fissure | 16 | 24–25 |
Secondary occipital sulci | 34 | 34 |
Sulci of the ventral cerebral surface | ||
Hippocampic fissure | 22–23 | |
Collateral sulcus | 23 | 27 |
Occipitotemporal sulcus | 30 | 33 |
Sulci of the lateral cerebral surface | ||
Superior frontal sulcus | 25 | 29 |
Inferior frontal sulcus | 28 | 29 |
Superior temporal sulcus (posterior part) | 23 | 27 |
Superior temporal sulcus (anterior part) | 32 | |
Inferior temporal sulcus | 30 | 33 |
Intraparietal sulcus | 26 | 28 |
Insular sulcus | 34–35 | 34 |
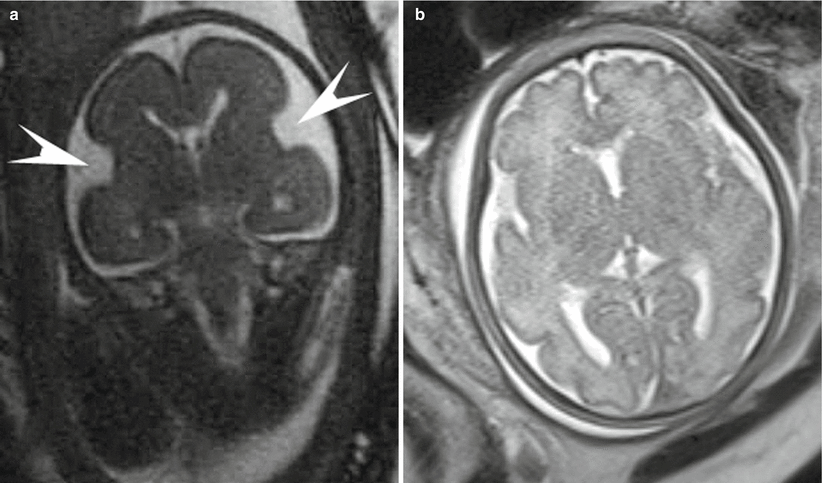
Fig. 3.5
Coronal SSFP (a) and axial (b) SSFSE images through the fetal brain in two different patients compare the sulcation pattern at different gestational ages. (a) shows the smooth cortex and early Sylvian fissure (arrowheads) formation at 24 weeks gestation. (b) highlights the complex cortical folding pattern in a near-term fetus
In addition to parenchymal folding, the size and morphology of certain parenchymal structures should be assessed. Tables 3.4, 3.5, 3.6, 3.7, and 3.8 and the associated figures can be used as a reference regarding size and location of measurement. Garel et al. provide reference data regarding additional parenchymal size landmarks [44]. Concomitant with sulcation and gyration, neural migration is occurring. At approximately 5 weeks post conception, the forebrain consists of two layers: a deep neuroepithelial layer (ventricular zone or germinal matrix) and a superficial layer (the preplate) [56]. Through complex radial migration, various layers of neural tissue are added to the developing parenchyma. The migration will give the appearance of distinct bands in the forebrain depending on the imaging time. For example, a multilayered appearance of the forebrain is typical between 23 and 28 gestational weeks [56, 57]. The following five distinct layers during these gestational weeks can be identified (from outside in): cortical plate, subplate zone, subventricular and intermediate zones, periventricular fiber-rich zone, and ventricular zone [57]. As neuronal migration begins, the germinal ventricular zone gradually regresses. By 29 weeks gestation, migration to the developing cortex is almost complete, and the ventricular zone disappears, resulting in the differentiation of only two layers: the cortex and the white matter. Persistent visualization of a multilayered appearance of the forebrain after 29 weeks signifies a migrational abnormality.
Table 3.4
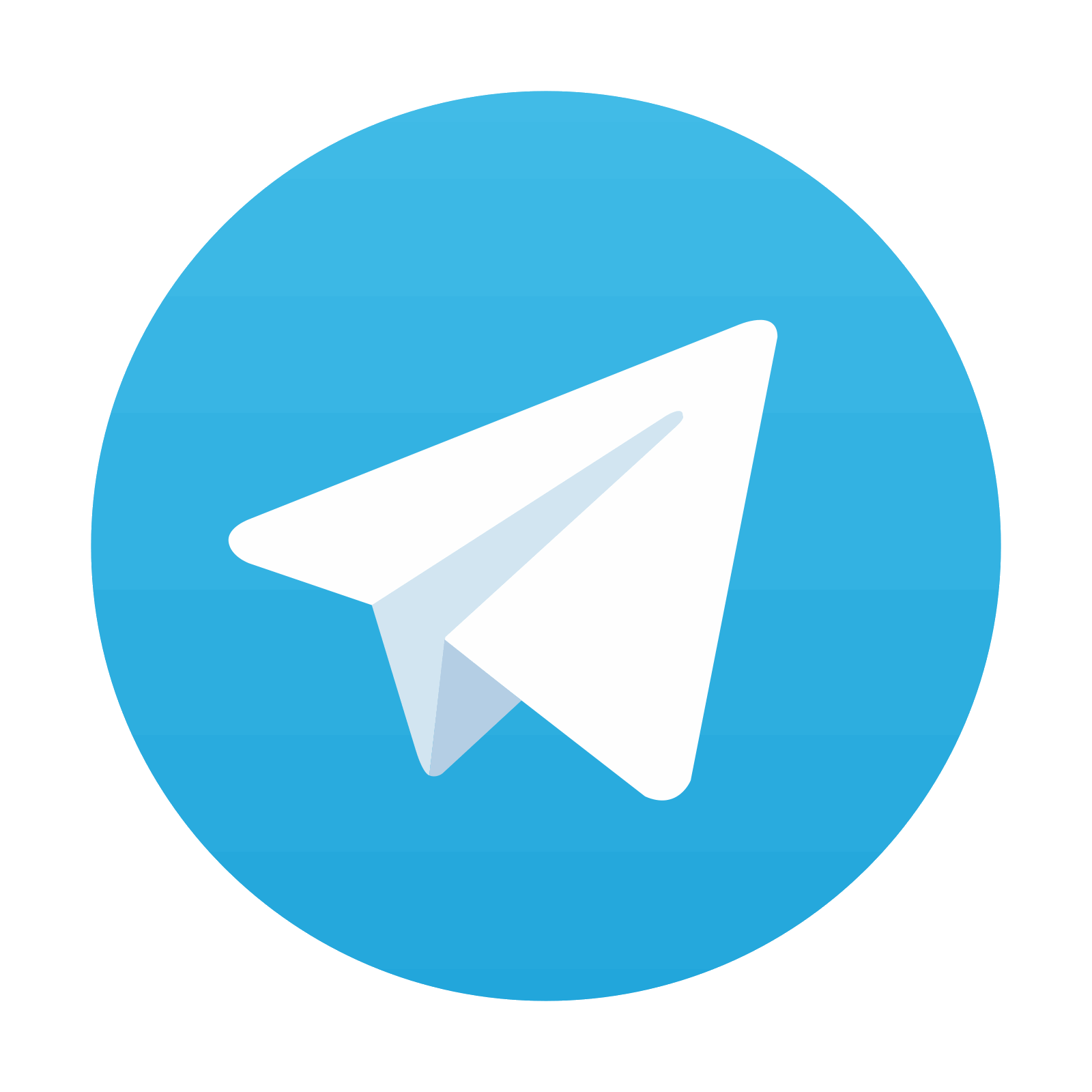
Fronto-occipital diameter (mm)
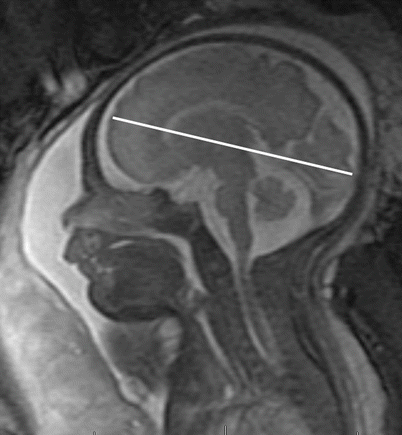
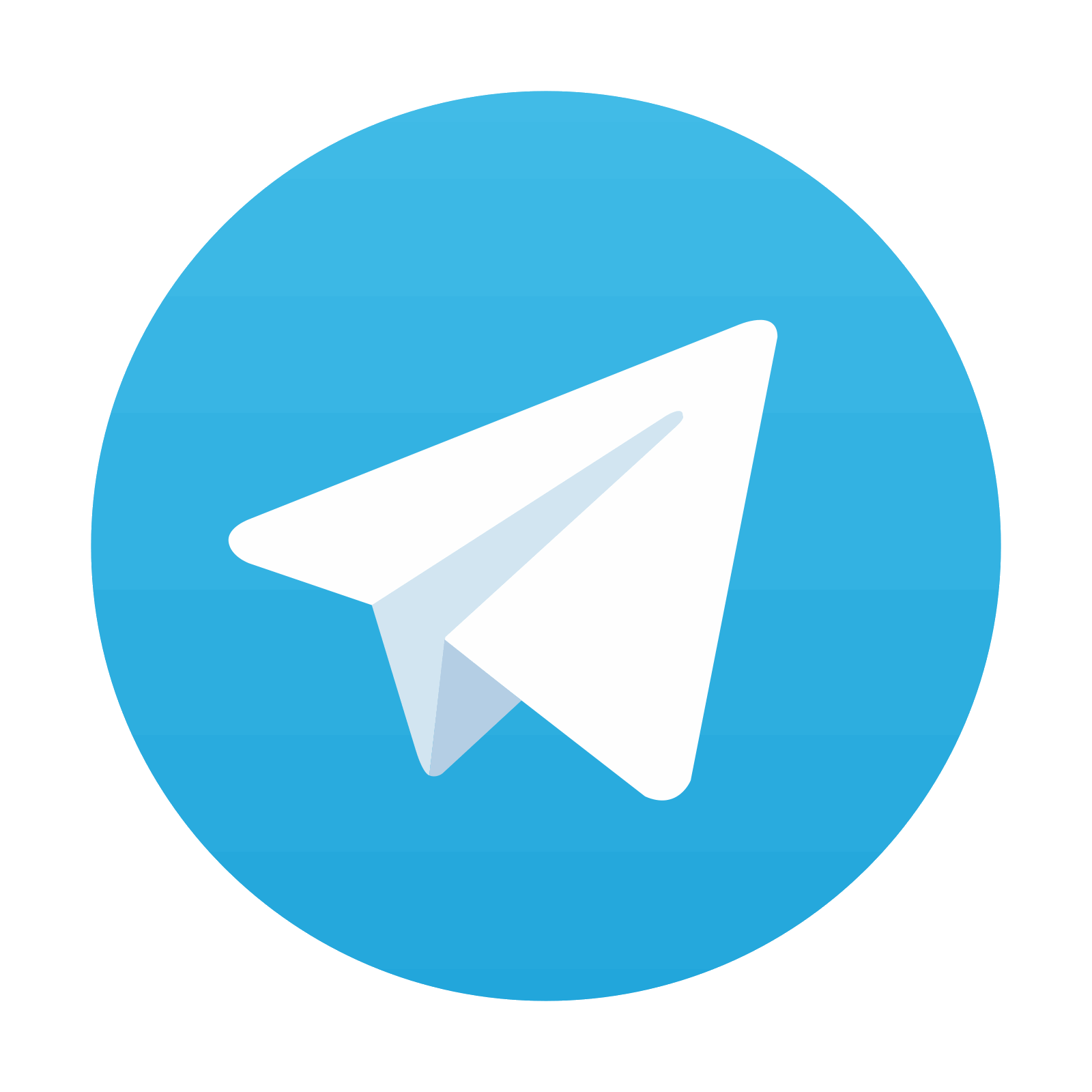
Stay updated, free articles. Join our Telegram channel
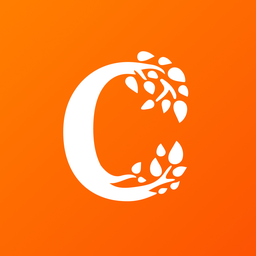
Full access? Get Clinical Tree
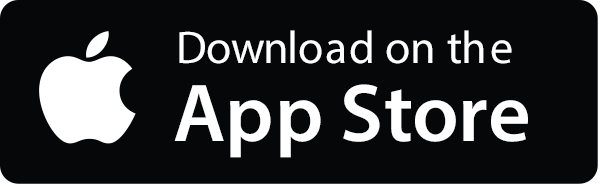
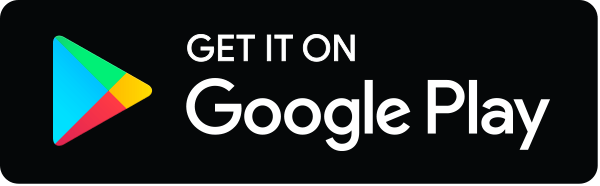
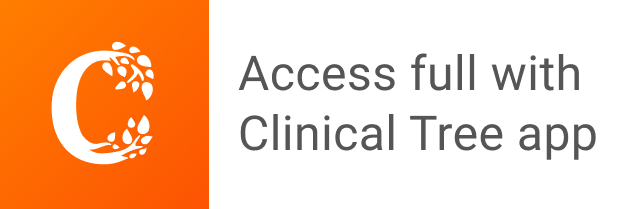