Isotope
T 1/2
β− MeV (%)
β+ MeV (%)
EC (%)
γ MeV (%)
18F
110 min
–
0.634 (97 %)
3 %
0.511 (194 %)
1.66 (3.1 %)
64Cu
12.7 h
0.573 (38.4 %)
0.655 (17.8 %)
43.8 %
0.511 (35.6 %)
1.35 (0.6 %)
68Ga
68 min
–
1.90 (87.7 %)
0.82 (1.2 %)
11 %
0.511 (178 %)
89Zr
78.4 h
–
0.902 (22.8 %)
77.2 %
0.511 (45.6 %)
0.909 (99 %)
124I
100.2 h
–
1.54 (11.7 %)
2.14 (10.8 %)
77 %
0.511 (45 %)
0.603 (63 %)
1.69 (10.9 %)
0.723 (10.4 %)
1.2 Chelation Chemistry
The most commonly used chelators for complexing 64Cu to nanoparticles are tetraazamacrocyclic ligands with pendant arms. The chelators DOTA (1,4,7,10-tetraazacyclododecane-1,4,7,10-tetraacetic acid), NOTA (1,4,7-triazacyclononane-1,4,7-triacetic acid), and TETA (1,4,8,11-tetraazacyclotetradecane-1,4,8,11-tetraacetic acid) have been the most widely used in 64Cu-labeled nanoparticles, with DOTA being the most popular (Fig. 1). Anderson and colleagues have shown that cross-bridged macrocycles form more kinetically stable 64Cu(II) complexes in vivo [2]; however, it has been demonstrated that there are only minimal differences in mouse biodistribution between DOTA and CB-TE2A conjugates of micellular nanoparticles [3]. Although the development of stable chelators for 89Zr is an active area of research [4, 5], thus far, the chelator deferrioxamine (DFO) is the chelator of choice for attaching 89Zr to nanoparticles. Examples of the nanoparticles labeled with radiometal chelates will be provided in the forthcoming sections.
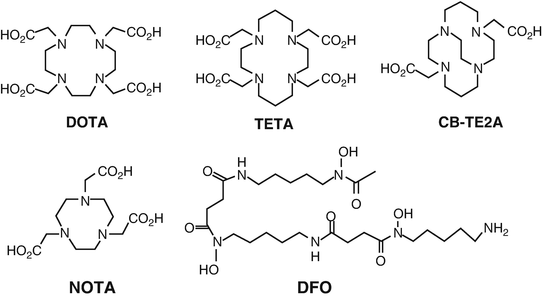
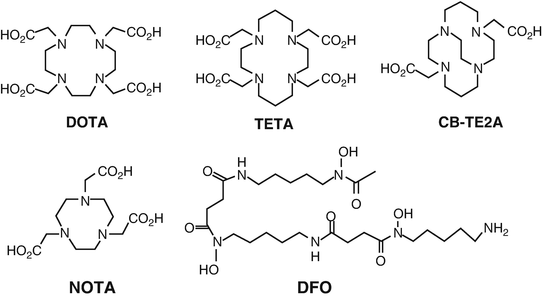
Fig. 1
Chelators that have been employed for complexing radiometals to nanoparticles
2 Silica-Based Nanoparticle PET Tracers
Silica-based nanoparticles that have been employed in PET imaging are typically modified forms of mesoporous silica or coated dense silica nanoparticles (dSiO2). Since the late 1990s, mesoporous silica nanoparticles (MSNs) have been studied extensively for a variety of applications due to their biocompatibility, large surface areas and ease of surface modification [6–9]. Various groups have coupled targeting moieties, drugs, and imaging agents to MSNs to investigate them as efficiency delivery vectors to tumors (Fig. 2) [10, 11].
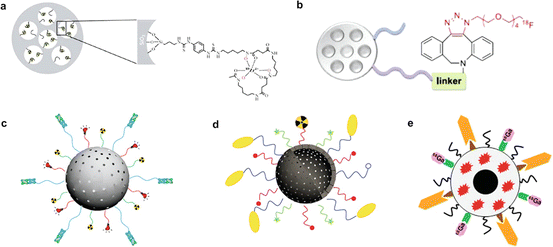
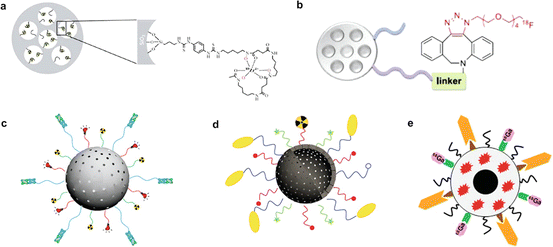
Fig. 2
Silica-based nanoparticles PET tracers : (a) representation of MSNs functionalized with APTMS, coupled with DFO-NCS and radiolabeled with radionuclide 89Zr [15]; (b) 18F-labeled peptide radiotracers [14]; (c) 64Cu-MSN-800CW TRC105(Fab) [16]; (d) 64Cu-NOTA-HMSN-fluorecein-PEG-cRGDyK nanoconjugate for drug delivery studies [18]; (e) 68Ga was labeled with the MF-uMUC-1 [17]
MSNs also experience the EPR effect when their diameter is 100–130 nm making them suitable for imaging tumors [12]. For example, aza-dibenzocyclooctyne (DBCO) PEGylated MSNs have been coupled via a biorthogonal in vivo click reaction to 18F-labeled azides to give 18F-DBCO-PEG-MSNs (~150 nm) (Fig. 2c) [13, 14]. This study investigated these PET agents in female nude mice bearing subcutaneously (s.c.) U87MG tumors (Fig. 3). The DBCO-PEG-MSNs were injected in mice 24 h before the 18F-labeled azide, allowing the MSNs to accumulate in the tumors prior to giving the 18F-azide, which would allow localization of 18F to the tumor, and clearance of all unreacted 18F-azide .
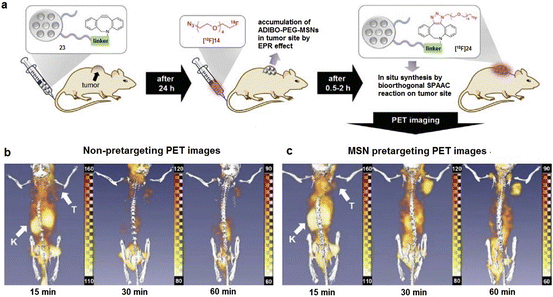
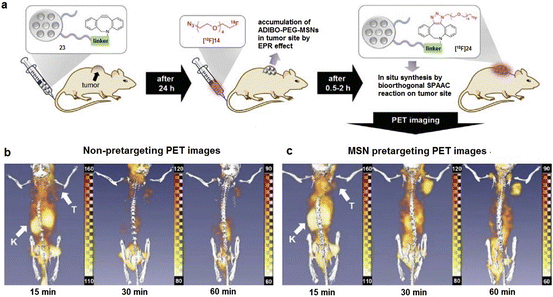
Fig. 3
Pretargeting PET imaging study by bioorthogonal covalent 18F-labeling. (a) The procedure for the in situ synthesis of 18F -DBCOT-PEG- MSNs in a living specimen by a bioorthogonal SPAAC reaction for the DBCO-PEG-MSN-pretargeting PET-imaging study. Three-dimensional reconstruction (upper) and transverse section (lower) combined PET-CT images of 18F-labeled azide ([18F]2 ; 2.6 MBq) in a U87 MG tumor-bearing mouse given only [18F]2 alone (non-pretargeted ; (b) or a mouse given DBCO-PEG-MSNs 24 h earlier (pretargeted ; c) recorded at 15, 30, 60, and 120 min after injection of [18F]2. T tumor, K kidneys [13]
PET images of the clicked MSNs in tumor were observed 2 h postinjection (p.i.) of the azide. Another group designed a new 89Zr desferrioxamine (DFO) MSNs (~180 nm) to image SCID mice with s.c. prostate carcinoma cell lines (LNCaP and C4-2) finding minimal dissociation of 89Zr4+ and typical biodistribution compared to other PET MSNs (Fig. 2a) [15].
MSNs can accommodate various surface modifications making them multimodality imaging agents not only for PET, but also near infrared fluorescence (NIRF) imaging and MRI. The surface of these nanoparticles can also be coupled to targeting agents. PET/ NIRF MSNs were developed with vasculature targeting capability to image 4 T1 murine breast cancer tumors in mice (Fig. 2d) [16]. Surface coupling of a human/murine chimeric IgG1 monoclonal antibody (TRC105(Fab)), NIR dye (800CW), and 64Cu-NOTA labeling resulted in MSNs (175.3 ± 9.7 nm) imaging tumors with 5.4 ± 0.2 % ID/g at 4 h p.i.. Another example of multimodality silica NPs was comprised of PET, NIRF, MRI agents and a targeting moiety that successfully imaged BT-20 cells in a nude mouse model (Fig. 2f) [17]. The silica-based nanoparticles (81 nm) have a cobalt ferrite core with rhodamine B in the silica shell and surface coupled underglycosylated mucin-1 antigen (uMUC-1 aptamer), along with NOTA for Ga-68 labeling.
Targeting of tumors has also been shown in hollow mesoporous silica nanoparticles (HMSNs) in U87MG tumor-bearing female athymic nude mice (Fig. 2e) [18]. Dense silica nanoparticles coated with MSNs have surface coupled cyclic arginine-glycine-aspartic acid (cRGDyK) peptide, 64Cu-NOTA as well as anticancer drug (Sunitinib) loaded in the core. PET images showed relatively high uptake (7.2 ± 0.6 % ID/g) at 0.5 h postinjection.
3 Gold Nanoparticle (AuNP) PET Tracers
Gold nanoparticles (AuNPs) are a promising platform for biomedical applications and have rapidly advanced toward multifunctional particles for imaging and treatment of cancer. AuNPs have been at the forefront of cancer research in recent years owing to the high biocompatibility via functionalization, their low toxicity of the gold core, and availability in a range of sizes and shapes [19, 20].
The readers are referred to a highly comprehensive review by Daniel and Astruc for information on the structure and properties of AuNPs [21]. Surface plasmon resonance (SPR) , a unique plasmatic absorption band phenomenon of AuNPs, can be converted to strong infrared spectral ranges, thereby allowing vital optical imaging in tissues where light exhibits minimal absorption and deep penetration in tissue. These specialized properties have also been used for photothermal therapy, which is a noninvasive, accurately targeted hyperthermia cancer treatment based on the optical absorbance of AuNPs in the intrinsic near-infrared (NIR) (650–900 nm) [22]. AuNPs also provide attractive scaffolds for many biomedical imaging modalities, including surface-enhanced Raman scattering (SERS) , two-photon photoluminescence (TPL) , magnetic resonance imaging (MRI) , positron emission tomography (PET) , and X-ray computer tomography (CT) imaging [23, 24].
3.1 Types of AuNPs
Typically, the dual imaging AuNPs are synthesized from commercial HAu3Cl4 by the reduction of Au3+ ions to metallic Au atoms (Au0) using citrate ions as a reducing and capping agent. There are different types of gold nanoparticles in the size range of 9–120 nm that have been developed with various shape, size, and physical properties (Fig. 4) [25].
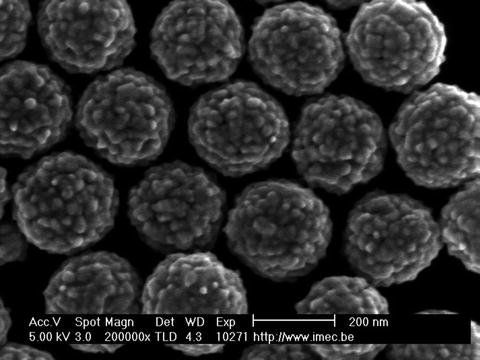
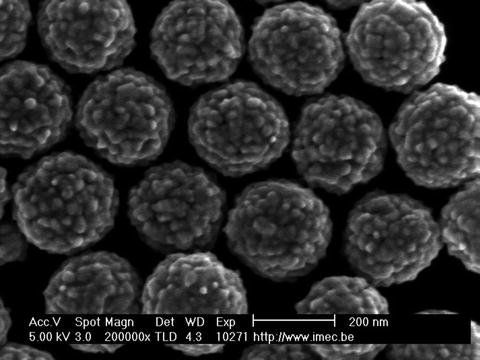
Fig. 4
SEM image of a monolayer portion showing self-assembled silica/gold nanoshells on a silane functionalized quartz substrate [25]
Gold nanosheres (gold colloids) in the size range of 2–100 nm can be produced by chemical reduction of gold chloride, with the properties controlled by citrate/gold ratio. The absorption spectra of gold nanospheres (visible range of 510–550 nm) are related to the size distribution [26–28].
Gold nanorods are synthesized by a special template method based on the electrochemical deposition of gold within the cylindrical pores of rigid matrices, such as nanoporous polycarbonate or alumina template membranes. Gold nanorods have absorption and scattering peaks that can be converted to the visible and near IR spectra, generating heat when excited by IR light. The characteristic has been widely applied to selectively destroy cancer cells [29].
Nanoshells , silica coated nanoparticles with a thin film of gold, have been used for an optical imaging with SPR in the visible to the NIR region [30, 31].
Gold nanocages are synthesized by reacting silver nanoparticles with chloroauric acid in aqueous conditions, and range in size from 10 to 150 nm. As the optical resonance peaks shift to near-infrared light, the strong absorption of gold nanocages enhances the contrast and photothermal effect for cancer diagnostic and therapy [32, 33].
3.2 Radiolabeling Chemistry of AuNPs
The surface of AuNPs can be modified with ligands, surfactants, polymers, and dendrimers. Upon functionalizing with thiol groups that strongly bind with gold, AuNPs can be readily conjugated to chelators or targeting molecules [34], enabling the conjugation of biofunctional chelates such as 1,4,7-triazacyclononane-1,4,7-triacetic acid (NOTA) or 1,4,7,10-tetraazacyclodedecane-1,4,7,10-tetraacetic acid (DOTA) via a thiol-maleimide coupling chemistry. Bifunctional chelators play an important role in conjugation of metal radionuclides on the AuNPs surface. For example, Gd3+ is attached on a chelate on the AuNPs surface for MRI imaging, whereas for PET imaging, radionuclides such as 64Cu, 68Ga, and 89Zr are generally used for PET/MR tracers. Targeting molecules added to the surface of AuNPs can render them tumor-specific for targeting via receptors expressed exclusively on target cancer cells, improving tumor localization over the nonspecific enhanced permeability and retention effect (EPR) [35].
64Cu-labeled RGD peptide-gold nanoshells (64Cu-NS-RGDfK) were developed for targeting integrin α v β 3 on tumor cells. The NSs were conjugated with RGDfK and DOTA through bifunctional PEG, and the surface modified size was ∼170 nm. PET/CT imaging of two rats bearing head and neck xenografts showed high tumor uptake beginning at 4 h postinjection and reaching a maximum at 20 h, with a decrease in tumor accumulation until 44 h postinjection [36].
Nonchelator conjugated [64Cu]CuS AuNPs (11 nm) was developed for a tumor theranostic probe both for PET imaging and as photothermal ablation agents using a passive targeting strategy in a breast cancer mouse model. At 24 h, biodistribution in mice bearing subcutaneous U87 glioma xenografts showed that the PEG-[64Cu]CuS NPs reduced liver/spleen uptake and enhanced tumor uptake ratio (7.6 ± 1.4 %ID/g) [37].
Other chelate-free 64Cu-labeled alloyed AuNPs (27 nm hydrodynamic (HD) size) were developed for cancer imaging . In these AuNPs, 64Cu is directly incorporated into the lattice of the gold nanoparticle structure, maintaining high stability in vivo. The PET/CT image using EMT-6 tumor-bearing mouse showed 4.93 ± 0.32 % ID/g of tumor uptake ratio at 1 h of postinjection, increasing at 48 h pi to 16.8 ± 0.98 %ID/g, with a tumor/muscle ratio of 16.2 ± 1.07 [38]. However, there was very high liver and spleen uptake of these particles at 48 h postinjection (~45 % ID/g liver and ~200 %ID/g spleen), (Fig. 5). The surface of these 64CuAuNCs was then PEGylated with different-sized PEG chains (64Cu-labeled AuNCs-PEG350, HD size of 4.3 nm vs. AuNCPEG1000, HD size of 6.9 nm) [39]. Biodistribution in PC3 tumor-bearing mice showed that both particles had dramatically decreased spleen uptake at 48 h (5 % ID/g or less), and the liver uptake was also <20 % ID/g. There was also significantly lower tumor uptake (3–5 % ID/g) for both sized AuNCs, with tumor:muscle ratios ~2.5 [38].
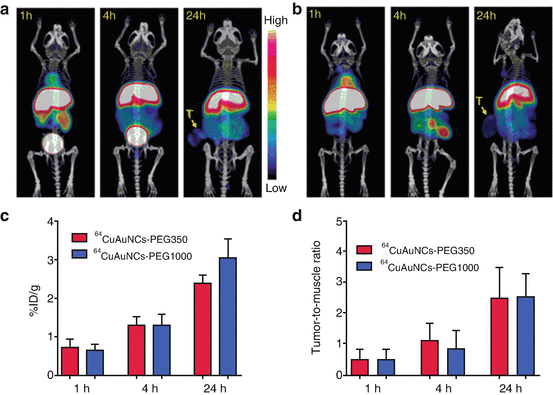
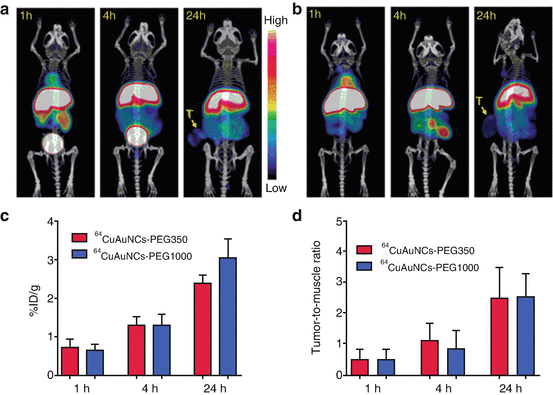
Fig. 5
PET/CT images at 1, 4, 24 h postinjection of 64Cu-AuNCs-PEG350 (a) and 64Cu-AuNCs-PEG1000 (b) in PC3 prostate-tumor-bearing mice. Quantitative tumor uptake (c) and tumor:muscle ratios (d) of the two agents (38)
64Cu-labeled hollow gold nanospheres (HAuNS) showed differential tumor uptake after hepatic intra-arterial (IA) and intravenous (IV) injection in VX2 liver tumor-bearing rabbits. RGD-PEG-HAuNS had an average diameter of ~40 nm. A DOTA analog chelator was attached to RGD-PEG-HAuNS for radiolabeling with Cu-64. PEG-HAuNS with lipiodol (IA-PEG-HAuNS-lipiodol) after hepatic intra-arterial injection had the highest tumor uptake (0.3319 ± 0.0711 ID%/g) compared to that of other 64Cu-labeled PEG-HAuNS and 64Cu radiolabeled RGD peptide-HAuNS after IA and IV injection, respectively. The result showed that adding iodized oil to PEG-HAuNS group can increase delivery of nanoparticles to hepatic tumors [40].
An RGD peptide was coupled with Gd-chelate coated gold nanoparticle (Au@DTDTPA-RGD) and labeled with 68Ga for a dual PET/MRI imaging modality targeting integrin α v β 3 receptor-positive U87MG cancer cells. Biodistribution studies showed that the tumor to muscle ratio increased from 1 to 2 h postinjection (3.71 ± 0.22 and 4.69 ± 0.09 respectively) [41].
89Zr-labeled anti-CD105 was coupled with gold nanoparticles (89Zr-anti-CD105-AuNPs-PPAA), having a mean diameter of 102.6 ± 4.0 nm. Two groups of B16 melanoma-bearing mice were injected with 89Zr-Df-Bz-NCS-anti-CD105 antibody or with 89Zr-anti-CD105-AuNPs-PPAA, respectively. The similar PET imaging pattern was observed, but 89Zr-anti-CD105-AuNPs-PPAA had higher uptake in liver, spleen, and lung compared to that of 89Zr-Df-Bz-NCS-anti-CD105 antibody due to its large size of the nanoparticle. The biodistribution study between 89Zr-anti-CD105-AuNPs-PPAA and 89Zr-Df-Bz-NCS-anti-CD105 showed that no significant differences in tumor:background ratios were observed [42].
4 Ultrasmall Nanoparticle PET Tracers
Ultrasmall nanoparticles are defined by their size (less than 10 nm), falling within the renal glomerular filtration size cutoff (~10 nm) [43–46]. Nanoparticles that fall between 10 and 200 nm are known to accumulate in the reticuloendothelial system (RES), which includes the liver, lymph nodes, bone marrow, and spleen, leading to longer retention in the body that could cause long-term adverse effects [47–50]. Small nanoparticles (~10–100 nm) and ultrasmall nanoparticles have unique properties, sparking interest in targeted therapies using PET imaging. Small and ultrasmall nanoparticles have provided biocompatible vectors compact enough to study metabolic pathways and therapies (Fig. 6). Some small nanoparticles mimic lipoprotein structures to sustain longer circulation as an alternative to PEGylation. A porphylipoprotein (PLP) nanoplatform (20.6 ± 5.2 nm) has been developed for fluorescence imaging and photodynamic light therapy of glioblastoma multiforme (Fig. 6a) [51]. PET imaging using 64Cu-labeled versions of PLP and PEGylated PLP in SKOV3 orthotopic ovarian cancer model showed similar uptake in tumors, but striking differences in spleen uptake, 3.4 ± 0.2 % ID/g versus 19.8 ± 1.6 % ID/g, respectively. Other compositions of similar sized nanoparticles like ultrasmall superparamagnetic iron oxide nanoparticles (USPIOs) have also been studied for their biocompatibility and cellular uptake effi ciency (Fig. 6b) [52]. USPIOs were coated with octylamine-modified polyacrylic acid (OPA) (10 ± 2 nm) and conjugated with a 64Cu chelator (DMPTACN) for PET imaging of mice bearing tumors of various human cell lines (epidermoid carcinoma A431, squamous cell carcinoma FaDu, ductal carcinoma MDA-MB 435S, umbilical vein endothelial HUVEC), finding generally low toxicity.
Nanodots also fall into the category of ultrasmall nanoparticles and have shown interesting applications as photothermal agents and in tumor imaging. Copper-64 labeled copper sulfi de nanodots ([64Cu]CuS NDs) coated with polyvinylpyrrolidone (~5.6 nm) were found to have tumor uptake in 4 T1 tumors in Balb/c mice (3.62 ± 0.50 % ID/g, 2 h p.i.) and fast renal clearance (Fig. 6c) [39, 53]. Cornell dots (C dots) are ultrasmall inorganic optical-PET imaging nanoparticles probes. The silica-based core contains encapsulated Cy5 fluorophore and the surface is PEG-coated with conjugated 124 I-labeled cyclic arginine-glycine-aspartic acid (cRGD, for αvβ3 integrin targeting) (Fig. 6d) [54]. The C dots were found to have high receptor-binding specificity, and biodistribution and targeting kinetics data, clearance and dehalogenation profiles, blood/tissue residence times, and bioavailability and radiation dosimetry were reported (Fig. 7). This ultrasmall nanoparticle has received FDA-approval for a first-in-human clinical trial and found to be safe in human (five patients) as a diagnostic for metastatic melanoma [55]. The preliminary trial found that the agent was well tolerated, cleared quickly from the body through the kidneys, and the 124 I-label was stable in vivo. Two patients showed tumor uptake in a pituitary and a liver lesion. To date, this is one of the few nanoparticle-based PET tracers to be evaluated in human cancer patients. Some ultrasmall nanoparticles are small enough to be considered clusters or matrices. One group has made chelator-free labeled [64Cu]Cu nanoclusters coated with bovine serum albumin (BSA) for PET imaging for tumors (Fig. 6e) [56, 57]. These nanoclusters have been conjugated with a tumor target peptide, lutenizing hormone releasing hormone (LHRH), giving [64Cu]Cu NC @BSA-LHRH (3.8 ± 0.5 nm) that were studied in mice bearing orthotopic A549 lung tumors (Fig. 8). The targeted nanoclusters were found to have much higher tumor uptake 12 % ID/g than nontargeted 3 % ID/g at 4 h p.i. Other interesting ultrasmall nanoparticles were made from a polysiloxane matrix coated with chelators for PET/MR dual imaging [58]. These ultrasmall nanoparticles (AGuIX) are only 2.5 ± 0.1 nm in diameter (Fig. 6f). They have been well-characterized and have shown no toxicity in U87MG (human primary glioblastoma) and HEKβ3 (human embyonic kidney) cell lines.
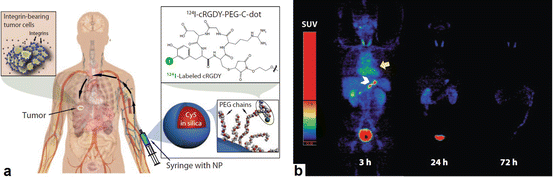
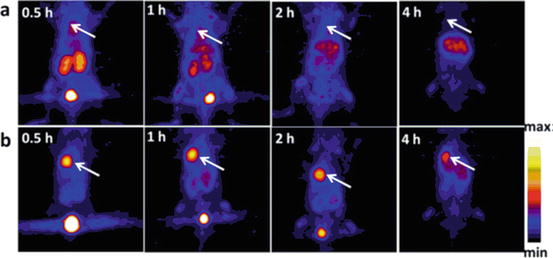
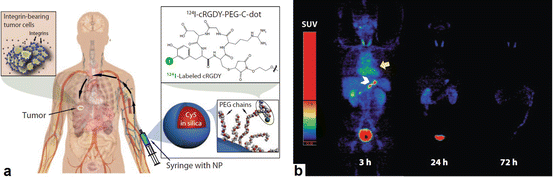
Fig. 7
(a) Cornell dots (C dots) conjugated with 124I-labeled cRGDY have been investigated in humans with cancer for safety, biodistribution, and dosimetry; (b) PET image in patient #3 showing activity in bladder, heart, and bowel [55]
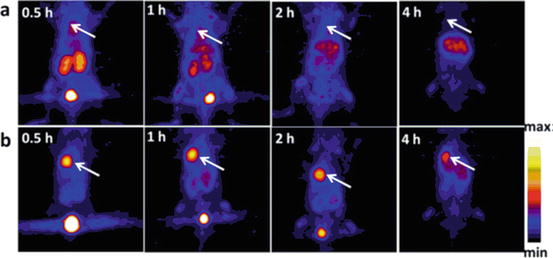
Fig. 8
Representative PET images of coronal single slices on orthotopic A549 lung tumor-bearing mice after intravenous injection of 6.7 MBq of [64Cu]CuNC@BSA (a) and [64Cu]CuNC@BSA-LHRH (b). Images were acquired at 0.5, 1, 2, and 4 h. White arrows indicate the lung tumor [57]
PET/CT imaging using 68Ga-labeled AGuIX was found to have rapid clearance and coherence with MR images. The development of ultrasmall nanoparticles is becoming a new avenue for targeted imaging and therapy.
5 Radiolabeled Iron Oxide Nanoparticles (IONPs)
Iron oxide particles have been widely studied as an excellent MRI contrast agent in clinical trials due to its ideal paramagnetic and low toxicity [59, 60]. MR imaging is optimized with functional parameters such as spin-lattice relaxation time (T1) and spin–spin relaxation time (T2), which are a function of the local chemical structure of the molecules being imaged. The core of iron oxide particles is composed of iron and oxygen atoms, generating mostly magnetite (Fe3O4), magnemite, (γ-Fe2O3), and hematite (α-Fe2O3), which exhibit superparamagnetic physical properties at ambient temperature if the core diameter is relatively small (<20 nm) [61–63]. Iron oxide nanoparticles (IONPs) have a number of advantages for multimodality (e.g., PET/MRI) imaging: 1) they can be modified on the surface for conjugation with a large number of targeting ligands, thus enhancing the biological specificity and affinity to targeted molecules; 2) the nanoparticles can be coupled with chelators for labeling with metal radionuclides for PET or SPECT imaging; and 3) iron oxide nanoparticles can circulate in the blood vessels for a relatively long time, moving larger amount of radionuclides or other cargo to targeted organs [64]. Please see Chap. 10 for more detailed information on structural properties of IONPs.
64Cu-DOTA-RGD-conjugated IONPs were developed for targeting tumor α v β 3 integrin. Polyaspartic acid (PASP)–coated iron oxide was coupled with RGD and DOTA via surface amino groups for 64Cu-labeling. The hydrodynamic diameter is 45 ± 10 nm. In vivo PET studies in U87MG tumor-bearing mice showed tumor imaging at 1 h (7.9 ± 0.8 %ID/g), 4 h (10.1 ± 2.1 %ID/g), and 21 h (9.8 ± 3.2 %ID/g), respectively, after injection of 64Cu-DOTA-iron oxide-RGD [65].
89Zr-Deferoxamine-RGD-IONP, another RGD-iron oxide, was developed targeting ανβ3 and ανβ5 integrins overexpressed in nascent endothelial cells during angiogenesis in various tumors, but not in inactive endothelial cells. The PET/CT and MRI imaging after intravenously injection in tumor-bearing mice showed high accumulation in the liver and spleen at 1 h after injection, which remained high at subsequent time points. After 24 h, accumulation in tumor was observed with persistent and intense signal until 72 h, clearly delineating the tumor [66].
Protein-based ligands, such as affibodies, have been investigated as tumor targeting agents. An anti-EGFR affibody was conjugated to Au-Iron Oxide NPs (NOTA-Au-IONP-affibody) having an average size 24.4 ± 2.0 nm, and was labeled with 64Cu. An in vitro study showed that NOTA-Au-IONP-Affibody probes had a higher cellular uptake in EGFR-positive tumor A431 cells at 1 and 2 h time points, respectively, compared with blocking samples (Fig. 9). The small animal PET images showed high tumor accumulation (4.6 % ID/g at 24 h p.i.), and high tumor-to-normal tissue contrast for 64Cu-NOTA-Au-IONP-affibody. The biodistribution studies also indicated that the nanoprobe had higher tumor uptake value compared to the blocking group [67].
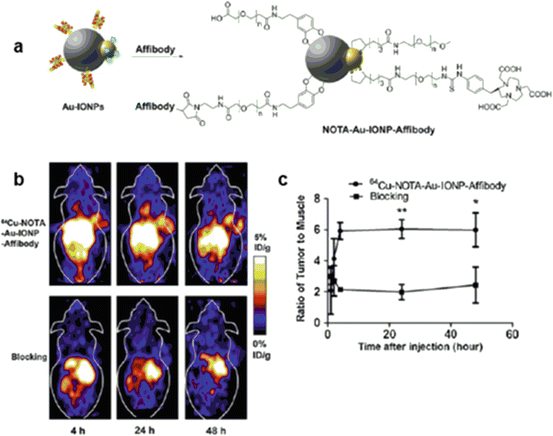
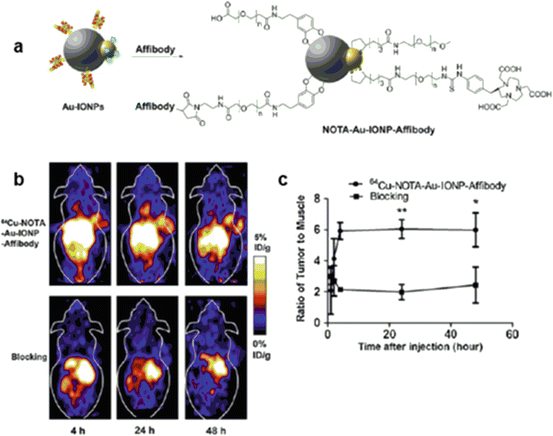
Fig. 9
(a) Schematic illustration of Affibody binding domain with Au-IONP and its functionalization and conjugation with affibodies and the NOTA chelator; (b) PET image of A431 tumor-bearing mice at 4, 24, and 48 h postinjection of 64Cu-NOTA-Au-IONP-Affibody, with and without blocking; (c) tumor:muscle ratios with and without blocking [67]
6 Liposome-Based PET Agents
Liposomes used in PET imaging are an attractive vector for imaging tumors and diseased tissues, as well as drug targeting. The study of liposomes as imaging agents started in the late 1980s [68], although it was not until the late 1990s that liposomes were radiolabeled for imaging purposes [69–72]. Generally, liposomes are lipid vesicles formed from thin lipid films or cakes. Upon hydration, layers of the bilayer sheets self-assemble into multilamellar vesicles (MLVs) where the hydrophilic portions face the water surrounding and within the vesicles. The size of these liposomes can then be reduced to unilamellar vesicles by input of either sonic energy (sonication) or mechanical energy (extrusion). Liposomes are typically characterized by their particle size (dynamic light scattering), zeta potential (laser doppler microelectrophoresis), and uniform size distribution. Like all nanoparticles used in medicine, the size and charge of the nanoparticle play a big role in their fate in vivo. Most liposomes range in size from 90 to 925 nm and are either negatively charged or neutral. This size range is meant to be above the renal threshold for longer circulation.
The success of liposomes in clinical and investigational research has led researchers to combine the physiological properties of liposomes with the quantitative imaging capability of PET. The longer-lived PET radionuclides (64Cu, 62Cu, 124I, 89Zr) have been typically investigated with liposomes to take advantage of the EPR effect; however, shorter-lived radionuclides (11C, 18F, 15O) have also been studied for PET imaging [73]. Incorporating a radionuclide for PET imaging into a liposome can be achieved by (1) encapsulation; (2) remote loading (after-loading); (3) bilayer chelation; or (4) surface chelation.
A 18F-labeled cholesteryl ether (18FCE) PEGylated liposome employed bilayer chelation to incorporate radioactive lipophilic labels in the liposome membrane (Fig. 11a) [74]. The liposome 18FCE was found to accumulate in NCI-H727 human lung carcinoid tumors of tumor-bearing nude mice after 8 h (2.25 ± 0.23 %ID/g). Another 18F-labeled liposome (18F-TCO-liposome) incorporated the fast tetrazine (Tz)/trans-cyclooctene (TCO) inverse electron demand Diels-Alder cycloaddition (IEDDA) and pH (low) insertion pepide (pHLIP) to speed up tumor accumulation (Fig. 11b) [75]. Tumor-bearing athymic nude mice were injected with SKOV3 ovarian cancer cells and pHLIP-Tz (Fig. 10). The 18F-TCO-liposome could be detected as early as 30 min after injection of the liposome. After 120 min, tumors marked with pHLIP-Tz had higher activity (3.5 ± 1.2 %ID/g) compared to tumor without pHLIP-Tz (0.46 ± 0.04 %ID/g).
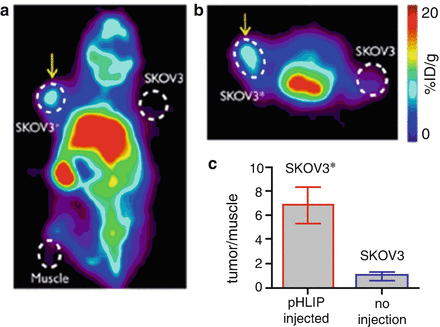
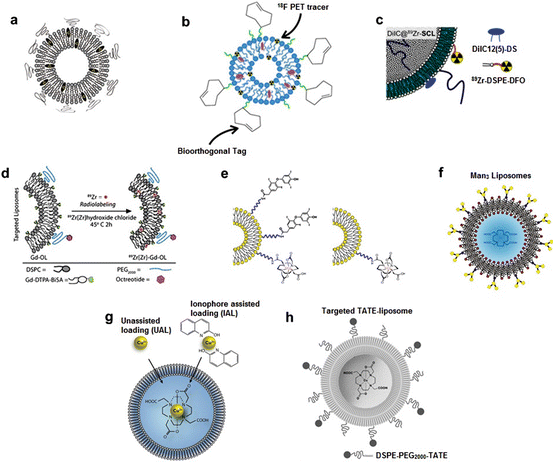
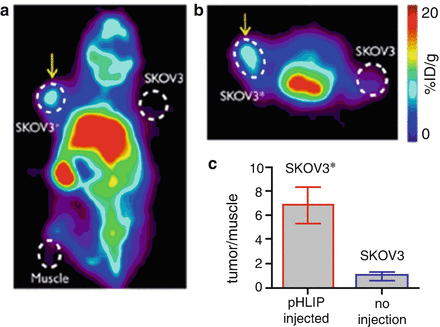
Fig. 10
Biodistribution and PET imaging of bioorthogonal 18F-liposomes as a selective marker for tetrazine-labeled SKOV3 ovarian cancer xenografts. (a, b) PET imaging at 2 h postinjection in a mouse bearing both pHLIP-Tz-treated (SKOV3, left shoulder, yellow arrow) and untreated (SKOV3 right shoulder) tumors. Normalized tumor:muscle ratios for treated and untreated tumors at 2 h post-injection [75]
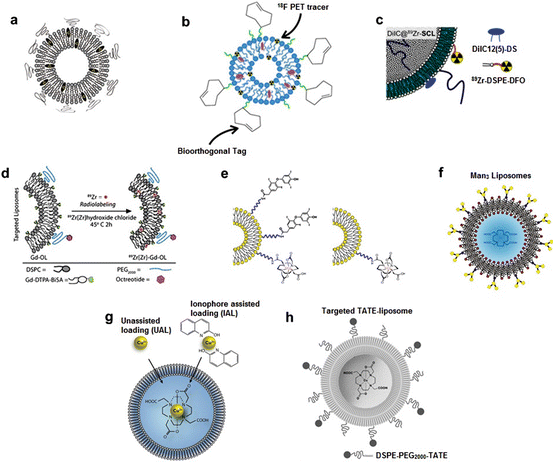
Fig. 11
Liposome-based PET agents : (a) PEGylated liposome incorporating radioactive lipophilic labels in the membrane [74]; (b) 18F-TCO-liposomes and pHLIP-Tz coupling for pretargeting of acidosis [75]; (c) dual-labeled liposome DiIC@89Zr-SCL [78]; (d) OCT was conjugated to preformed Gd-Control LPs (CL) resulting in targeted OCT-LP (OL) [80]; (e) tetrac/64Cu-DOTA-liposome (left) and 64Cu-DOTA-liposome (right) [85]; (f) Mannose liposomes for remote loading of the 64Cu-DOTA [84]; (g) Loading of 64Cu2+ into liposomes [82]; (h) PEGylated (DSPE-PEG2000) targeted TATE-liposome [83]
For longer circulating PET imaging agents, Zr-89 is favored due to its half-life (78.4 h) and relatively lower fraction of gamma radiation than 124I and 86Y [76, 77]. Several groups have utilized the combination of the liposome’s EPR effect over time and 89Zr as a PET imaging agent. 89Zr-PEGylated-liposomes radiolabeled by surface chelation have shown tumor accumulation peaks at 24–48 h postinjection (Fig. 11c) [78, 79]. The PEG groups on the surface of the liposomes assist with longer circulation, giving the liposomes time to accumulate in/around tumors. Another 89Zr-PEGylated-liposome also included a targeting agent, octreotide, on the surface of the liposome (OL) (Fig. 11d) [80]. Octreotide is a peptide targeting human somatostatin receptor subtype 2 (SSTr2) found on tumor cells. The 89Zr-Gd-OL imaging agent was found to specifically accumulate at the tumor site at 50 h postinjection.
Other radionuclides , especially Cu-64, have gained attention as a viable radionuclide coupled to liposomes for PET imaging. Several groups have used Cu-64 with their liposomes to study a variety of tumor models. Remote loading and surface chelation are some of the more common methods for radiolabeling liposomes. Remote loading using 2-hydroxyquinoline has been shown to be highly efficient compared to other ionophores [81]. Unassisted radiolabeling or remote loading without the use of ionophores has shown evidence of similar radiolabeling efficient compared with ionophores (Fig. 11f) [82]. Copper-64 has been remotely loaded into liposomes containing surface targeting SSTr2 in human neuroendocrine carcinoma in mouse models (Fig. 11g) [83] and mannose-coated liposomes targeting macrophages in the tumor microenvironment (TME) [84]. Surface chelation and modification using 64Cu has yielded informative findings in tumor targeting and organ uptake. DOTA and TETA macrocycle analogs have been used to coordinate the radionuclides to the liposome giving a stable radiolabeled liposome (Fig. 11e) [85, 86].
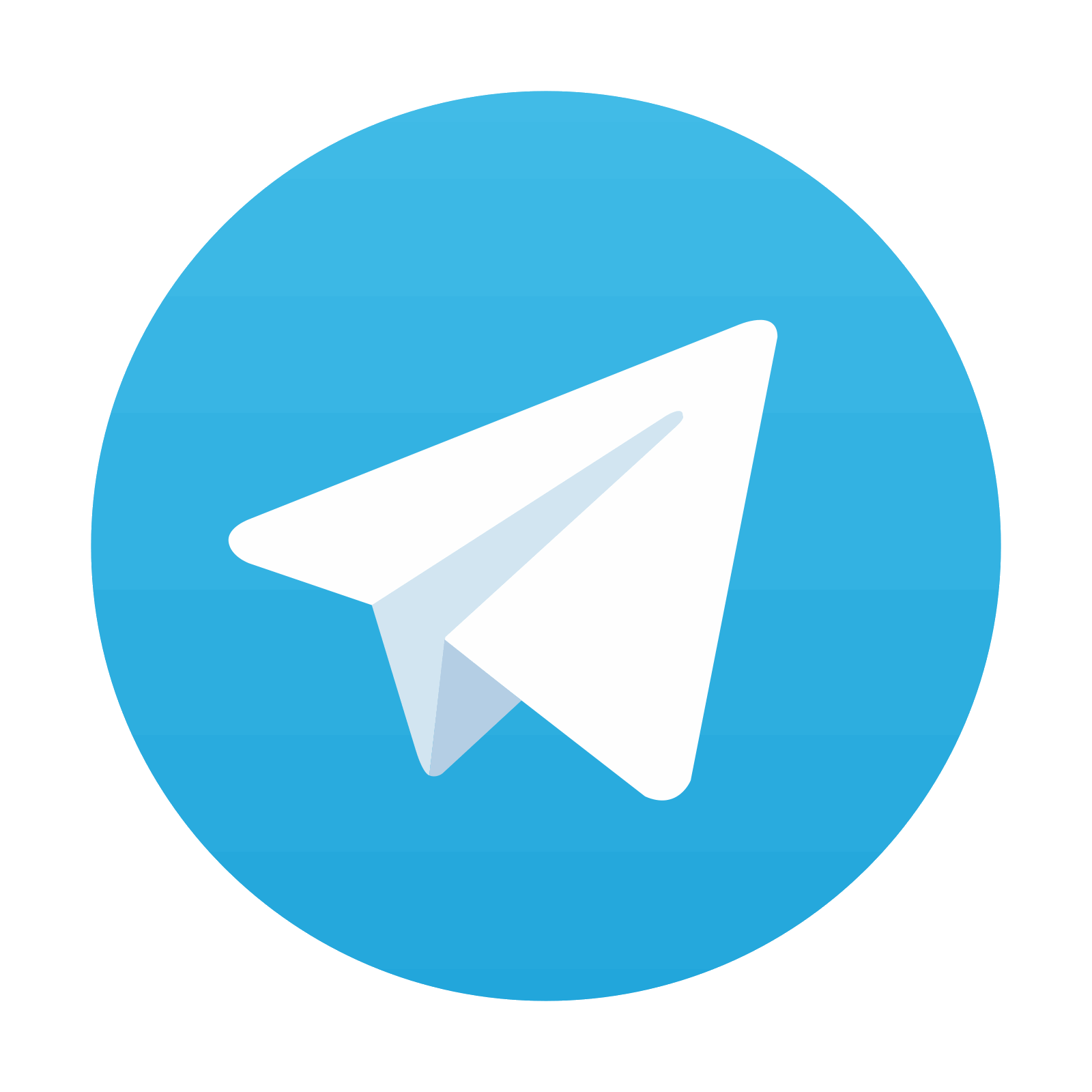
Stay updated, free articles. Join our Telegram channel
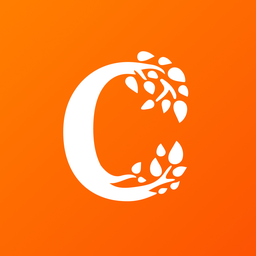
Full access? Get Clinical Tree
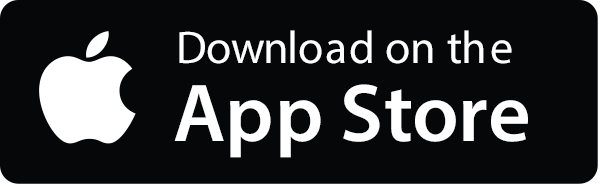
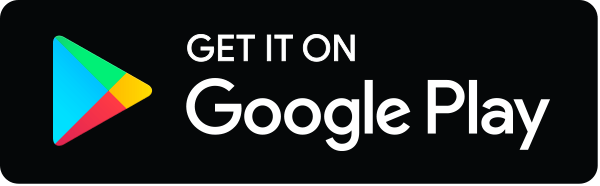