(1)
Where P is the photoacoustic signal intensity, F is the fluence of laser energy in mJ/cm2, μ a is the optical absorption coefficient of the absorptive media in cm−1, and γ is the Grüneisen parameter, which describes the heat transfer characteristics of the media. Therefore, photoacoustic signal is linearly dependent on the laser fluence irradiated onto the sample and how absorptive the sample is. Generally, two conditions must be met for the effect to occur, namely thermal and stress confinements. Thermal confinement is met when the duration of the light pulse is shorter than the thermal relaxation time of the media, meaning the pulse duration must be shorter than the time it takes for the heat generated by absorption to dissipate. Stress confinement is met when the pulse duration is shorter than the stress relaxation time. Most laser pulses less than 10 ns will fulfill these requirements for photoacoustic imaging purposes.
2.1 Spectroscopic Photoacoustic Imaging
In biological tissue, the dominant optical absorber is hemoglobin in its two oxygenation states (see Fig. 1). As photoacoustic imaging contrast is highly dependent on optical absorption, this implies photoacoustic imaging is inherently suited for imaging blood. Several applications take advantage of this property such as imaging of tumor neoangiogenesis, monitoring antiangiogenic therapy, and imaging of ischemic events, to name a few [7–11]. However, examining other endogenous or exogenous absorbers beyond hemoglobin states, which is crucial to expand the cancer imaging application of photoacoustic imaging, can result in low signal to background ratios, as optical absorption of hemoglobin will dominate the photoacoustic signal production. One solution is to compare pre- and post-injection images of contrast agents to display signal only corresponding to the exogenous agents. However, using subtraction of images to remove background hemoglobin signal is prone to error due to physiological changes including tumor growth, changes in blood flow, and experimental setup, including laser fluence variations and imaging plane shifts [12]. Therefore, methods are needed to overcome these challenges and minimize background signal from hemoglobin when examining other endogenous or exogenous agents.
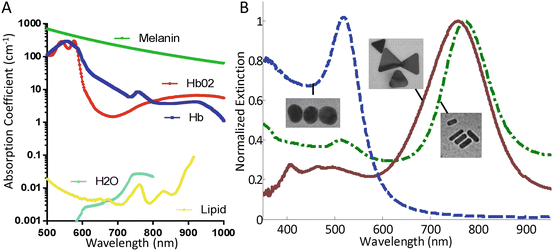
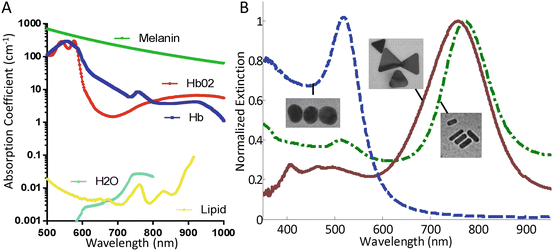
Fig. 1
Optical absorption of endogenous chromophores and exogenous nanoparticle contrast agents. (a) Absorption coefficients of several endogenous tissue chromophores. Melanin has the strongest optical absorption, but only occurs in the skin, and therefore hemoglobin in its two oxygenation states (HbO2—oxygenated hemoglobin, and Hb—deoxygenated hemoglobin) dominates photoacoustic images over lipid and water imaging. These spectra have local minimums in the 700–900 nm range, where (b) various nanoparticles have tunable peaks in their optical absorption including silver nanoplates (brown line) and gold nanorods (green line). Nanospheres (blue line) act as precursor nanoparticles to synthesize anisotropic shapes
Spectroscopic photoacoustic imaging can be implemented to reduce the photoacoustic signal from blood and enhance the signal from the desired absorber by modeling images as linear combinations of absorbers. Optical absorption is dependent on the wavelength of light and the concentration of the photoabsorber . The absorption coefficient μ a(λ,r), which is dependent on the wavelength “λ” and the spatial position “r,” is defined as the product of the molar extinction coefficient (Ɛ) and the concentration (C) of the photoabsorber as described [12, 13], providing the following Eq. (2):

Assuming the optical absorption that provides photoacoustic signal is proportional to the sum of the n photoabsorbers within the imaging plane, the localized absorption coefficient μ a can be considered as the sum of the “n” individual absorption coefficients. Therefore, if at least “n” wavelengths are used to image a given plane, regression methods can be used to determine the relative concentrations of the “n” photoabsorbers in relative but arbitrary units within the region-of-interest based on the assumption that the photoacoustic signal (known variable as measured in the experiment) is proportional to the molar extinction coefficient “Ɛ” (a known variable from the literature [14, 15]) times the concentration “C” of the individual photoabsorbers (unknown variable). The power of the regression improves with additional wavelengths and/or those that represent salient spectral characteristics of the photoabsorbers [13]. The method is most accurately applied when examining a mixture of photoabsorbers that have (1) relatively good optical absorption with hemoglobin being the standard; (2) distinctive spectral characteristics such as sharp peaks; and (3) absorption spectra that are highly distinguishable from each other.

(2)
2.2 Label Free Spectroscopic Photoacoustic Imaging in Cancer
Endogenous or label-free photoacoustic imaging, involves the examination of intrinsic tissue constituents such as hemoglobin, lipid, melanin, and water, which can be selectively imaged using spectroscopic photoacoustic imaging due to their strong absorption characteristics in the near infrared light region [15–19]. In cancerous tissue, angiogenesis leads to formation of new vasculature, thereby contributing to significant changes in concentration of hemoglobin within the tissue compared to healthy tissue with a subsequent increase in photoacoustic imaging signal [20, 21]. A multitude of studies taking advantage of intrinsic photoacoustic imaging contrast obtained from differences in the absorption spectra of tissue absorbers, such as oxygenated and deoxygenated hemoglobin, have been reported in the literature [22–27]. In a recent study [28], it has been demonstrated that as many as four different breast histologies, including normal (n = 82), hyperplasia (n = 12), ductal carcinoma in situ or DCIS (n = 96), and invasive breast carcinoma (n = 61), can be differentiated with reasonable accuracy based on the photoacoustic imaging contrast obtained from oxygen saturation, total hemoglobin, and lipid content in a transgenic mouse model (27 mice, n = 251 mammary glands). With the help of a multiparametric analysis, it was shown that oxygen saturation performed best to differentiate clinically non-actionable (normal/hyperplasia) versus clinically actionable (DCIS and invasive breast carcinoma) findings. Furthermore, a reduction in lipid content was seen as the fat tissue being progressively replaced by a solid tumor during breast cancer development. However, the total hemoglobin was observed to be lower in DCIS and invasive breast carcinoma compared to normal and hyperplasic mammary glands in that study despite the general increase of vascularity in tumors. Therefore, due to complex biological processes, elucidating information concerning disease state based on those parameters only may be challenging.
Also, in instances where the blood vessels are still smaller compared to the detection sensitivity of the photoacoustic imaging system, which might often be the case in the early stages of cancer development, the intrinsic endogenous contrast may not be sufficient to detect any changes between cancer and surrounding healthy tissue. Moreover, not all cancers behave similarly, making it hard to estimate the type of change one might expect from the endogenous absorbers. For instance, will the concentration of lipid in cancer be more, or less compared to healthy tissue? Will different types of cancer show similar traits in absorption? Furthermore, the photoacoustic image contrast obtained from endogenous absorbers is not specific to the cancer or any other disease and may not be sufficient to provide a definitive diagnosis. As such, exogenous contrast agents , which can be synthesized to achieve a desired absorption, can be helpful in increasing the sensitivity and contrast-to-noise of photoacoustic imaging for cancer imaging. In addition, exogenous photoacoustic imaging agents, such as nanoparticles , that can interact with the tissue or bind to a cancer-specific biomarkers can also improve specificity, thereby allowing molecular imaging capabilities.
3 Nanoparticles for Photoacoustic Cancer Imaging
Nanoparticles are well suited for use as contrast agents, as their size is on scale with biological processes and cells, allowing visualization of the intricate processes that occur. These small sizes allow enhanced optical absorption, biodistribution, and clearance properties that can all be fine-tuned for specific applications. In the following sections, a brief introduction on general synthesis and bioconjugation methods of nanoparticles will be presented followed by key examples of use for photoacoustic imaging of cancer .
3.1 Plasmonic Noble Metal Nanoparticles
Plasmonic noble metal (e.g., gold, silver) nanoparticles constitute the most commonly researched probes for photoacoustic imaging of cancer . These particles have relatively facile and rapid (a few hours) synthesis methods with tunable size and shape parameters to allow for application-specific optimization of absorption properties that provide sharp absorption peaks within the near infrared tissue “optical window,” where endogenous tissues absorption is dominant otherwise. Furthermore, noble metal nanoparticles are easily bioconjugated to targeting moieties. However, in the case of gold nanoparticles, while they have little interaction with biological systems preventing toxicity, they are often retained in the liver and spleen. In the case of silver, which does degrade in biological environments, there is concern of potential silver toxicity . Current research is focused on overcoming these limitations because the per particle optical absorption tends to be higher in noble metal nanoparticles compared to any carbon nanotubes , polymer, or dye based nanoparticles due to the interesting phenomenon of plasmon resonance, making them promising contrast agents for photoacoustic imaging of cancer.
3.2 Plasmon Resonance
Nanoscale particles have many interesting properties that the same materials in bulk do not. One of the main reasons for this is the shift in the ratio of surface atoms to interior atoms. Surface atoms interact with the surrounding environment while interior atoms do not. This increased interaction with the environment gives rise to the superior optical absorption of noble metal nanoparticles because of surface plasmon resonance (SPR) [29–31]. Concretely, free electrons in metals are able to travel throughout the material, and in nanoscale particles, they do so free of scattering as the mean free path is greater than the size of the nanoparticle. Therefore, when an electromagnetic wave front with a wavelength larger than the diameter of the particle passes, the free electron cloud is polarized to the sides of the nanoparticle depending on the oscillating charge of the wave, resulting in coherent resonation at the same frequency. The size, shape, and surface environment of the nanoparticle all alter the SPR resonance (absorption) peaks, allowing nanoparticles to be finely tuned to specific applications in the visible and near infrared regions [29–31]. Once noble metal nanoparticles have the desired absorption characteristics, it is important to fine tune the pharmacokinetics and distribution for an optimal imaging system.
3.3 Bioconjugation
Due to their small size, unconjugated nanoparticles can be directly used as cancer contrast agents through the “enhanced permeability and retention effect” (EPR) which typically allows up to 5 % of the injected dose to accumulate nonspecifically within tumor tissues [32]. In tumors, the recruitment and growth of new blood vessels to supply nutrients occurs more quickly than typical angiogenesis [20]. Therefore, the organization and function of these rapidly grown vessels is abnormal, including poorly aligned endothelial cells, which leave large gaps of up to 800 nm in diameter between cells. Combined with ineffective lymphatic drainage, these factors make nanoparticles more likely to accumulate within tumor tissue compared to normal tissue [33, 34]. However, the efficiency of nanoparticle delivery using the EPR effect is highly variable between and within cancer types and stages, and is increased in preclinical xenograft tumor models due to a faster tumor development rate compared to native cancers [33]. Therefore, it is advantageous to conjugate molecular targets (such as antibodies, antibody fragments, peptides, aptamers, or metabolites [35]) to the surface of nanoparticles to, for example, increase receptor-mediated endocytosis into tumor cells or to allow for photoacoustic molecular imaging of a specific biomarker of the tumor vasculature. While other binding motifs (i.e., hydrophobic, electrostatic interactions, and van der Waals forces [36]) can be used to attach biomolecules to the surface of nanoparticles, covalent binding between SH, O, or N atoms through their unshared election pairs are preferred for clinical applications due to an increase in the stability of the constructs [36].
One of the typically leveraged bonds is the metal–thiol covalent bond mediated through sulfhydryl (SH) functional groups in thiols (R-SH), which is almost as strong as the gold–gold (Au–Au) bond, though the exact mechanism is still being elucidated [37, 38]. For bioconjugation purposes, the thiol interaction allows binding with cysteine amino acid residues, which have a thiol functional group allowing for direct protein conjugation. However, the number of cysteines can be limited or nonexistent in proteins, and therefore, proteins containing -NH2 groups (typically the more abundant lysine residue, occasionally arginine) could be attached by conversion to SH groups through EDC (1-ethyl-3-(3-dimethylaminopropyl) carbodiimide) or sulfo-NHS chemistry [35]. These reactions are fairly straightforward and short in duration up to a couple of hours [35]. Finally, to avoid a rapid immune response and clearance of nanoparticles triggered by nonspecific protein absorption on the surface, increased circulation time, and increased serum solubility [39], nanoparticles are typically coated with polyethylene glycol (PEG, PEGylation) using commercially available PEG-SH.
3.4 Gold Nanoparticles
Gold nanoparticles are by far the most commonly used plasmonic noble metal nanoparticle for photoacoustic imaging of cancer . Gold nanoparticles are bioinert in terms of cytotoxicity, but accumulate within the mononuclear phagocyte system [40], including the spleen, liver, and lymphatic system for up to 2–6 months (the final time point in several studies [41]); therefore, clinical use of gold nanoparticles remains elusive, as it requires more extensive biosafety and evaluation standards to elucidate whether this accumulation poses health risks, which is currently unclear. Extensive research has been dedicated to synthesizing monodisperse gold nanoparticles in a variety of sizes and shapes (spheres, rods, cubes, plates, barbells, rice, tetrapods, etc. see Fig. 2 [28, 42–44]), which allows fine-tuning of optical absorption properties for applications of photoacoustic imaging of cancer.
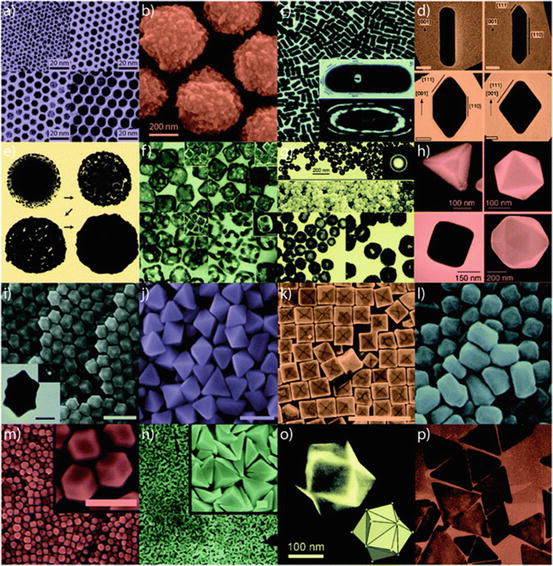
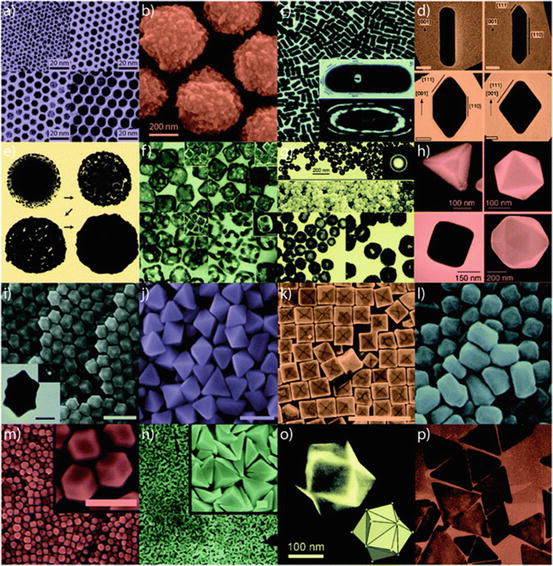
Fig. 2
Gold nanoparticles of various size and shape with potential applications in biomedicine. Small (a) and large (b) nanospheres, (c) nanorods, (d) sharpened nanorods, (e) nanoshells, (f) nanocages/frames, (g) hollow nanospheres, (h) tetrahedra/octahedra/cubes/icosahedra, (i) rhombic dodecahedra, (j) octahedra, (k) concave nanocubes, (l) tetrahexahedra, (m) rhombic dodecahedra, (n) obtuse triangular bipyramids, (o) trisoctahedra, and (p) nanoprisms [44]—Reproduced by permission of The Royal Society of Chemistry
3.4.1 Synthesis
While “top-down” approaches (starting with bulk gold and removing material until nanoparticles remain) exist for synthesis of gold nanoparticles, “bottom-up” approaches (building nanoparticles from reduced metal ions) have become sufficiently optimized to produce relatively monodisperse particles in bulk and will be the focus here. One common method for gold nanosphere production involves reduction of gold(III) derivatives, such as HAuCl4, using a weak reducing agent such as citric acid [45, 46]. The ratio of the reducing agent to the salt and reducing time determine the size of the nanoparticles, which are citrate stabilized in the solution. Particles can then be surface capped through PEGylation for purification and used for in vivo applications. Synthesis of anisotropic nanoparticles, most notably nanorods, begins with a similar method but using stronger reducing agents, (such as sodium borohydride) to produce small gold nanospheres (~4 nm). These small spheres act as “seeds” onto which carefully controlled epitaxial gold ion deposition is continued through the use of selective growth restriction surfactants that bind to faces of the seed with preferential crystal structures and atom spacing. In the case of gold nanorods, cetyltrimethylammonium bromide (CTAB) is used in combination with silver nitrate to block gold deposition on the {111} or {100} side faces, leaving the {110} common axis faces free for anisotropic growth {denote atomic crystal lattice structures} [47]. Similar to spheres, nanorods, and other anisotropic shapes can be stabilized through PEGylation for in vivo applications. Many other shapes (see Fig. 2) can be synthesized by varying synthesis parameters including temperature, component ratios, and surfactants. Using these synthesis methods combined with bioconjugation of targeting moieties allows fine tuning of nanoparticle composition for specific applications of photoacoustic imaging of cancer .
3.4.2 Applications
The use of nanoparticles as contrast agents for photoacoustic imaging of cancer currently remains in the preclinical research stage, though several interesting studies have been explored. For example, gold nanorods have been used to image multiple types of cancers. Jokerst et al. [48] demonstrated the use of gold nanorods to image three types of ovarian cancer tumors: 2008, HEY, and SKOV3 subcutaneously grown in mice (n = 3). They reported a 2.3-fold photoacoustic signal increase with intratumoral injection and a 3.5-fold increase with intravenous injection of gold nanorods at excitation wavelength of 756 nm respectively. The work by Zhong et al. [49] further demonstrates the applicability of gold nanorods beyond imaging, as they reported photoacoustic therapy using gold nanorods conjugated with folic acid in mice bearing HeLa (human cervical cancer) tumors that overexpress folate receptor. Folic acid conjugation allows the nanoparticles to bind to folate receptor whose expression is minimal in healthy tissues and high in cancers such as lung, breast, kidney, brain, cervix, or ovary cancer [50, 51]. Photoacoustic therapy although similar in principle to photoacoustic imaging , destructs individual target cells with laser-induced shock waves. When a photoabsorber is irradiated with a pulsed laser, the optical energy is transformed into mechanical energy, resulting in a shock wave without the side effect of heating or cavitation [52]. In photoacoustic therapy, the generation of shock waves rely on the efficiency of photoabsorbers to convert light energy into acoustic energy, with suitable candidates reported as indocyanine green containing nanoparticles (ICG-PL-PEG), single-walled carbon nanotubes , and gold nanorods [49, 53, 54]. When compared with images of mice injected with folic acid conjugated single-walled carbon tubes, and unconjugated gold nanorods, the tumor growth rate in mice treated with folic acid-conjugated gold nanorods was observed to be significantly lower over a duration of 24 days after treatment.
Another type of gold nanoparticles, nanoshells, have been used to study colon cancers. Li et al. [55] reported the intravenous use of PEGylated gold nanoshells in mice bearing CT26.wt colon cancer tumors grown subcutaneously. When imaged for 6 h using photoacoustic microscopy, the gold nanoshells were seen to be progressively taken up by the tumor foci, while those in the vessels were cleared out, indicating an enhancement in photoacoustic signals with a contrast ratio of 6.5 between the tumor foci to the vessels. Li et al. [56] reported the use of gold nanocages for photoacoustic imaging of cerebral cortex, sentinel lymph nodes, and melanoma in small animals. When gold nanocages were injected in rats to study their brain cortex [57], 81 % enhancement in blood absorption was reported over the intrinsic contrast. In a similar study conducted in rats to image sentinel lymph nodes [58], a gradual accumulation of gold nanocages was observed leading to a gradual increase in photoacoustic signal 28 min after injection. Likewise, gold nanocages functionalized with [Nle4, D-Phe7]-α-melanocyte-stimulating hormone were used for active targeting and resulted in a 300 % higher photoacoustic signal enhancement because of ligand-receptor binding on melanoma cells in mice compared to PEGylated gold nanocages 6 h after injection [59]. In a recent study by Shujing et al. [60], molecular imaging and photoacoustic therapy of gastric cancer stem cells using bioconjugated gold nanostars was reported. Gold nanostars conjugated with CD44v6 monoclonal antibody were intravenously injected in orthotopic and subcutaneous mice tumor models of human gastric cancer. Their results suggest that the bioconjugated gold nanostars successfully targeted the gastric vascular system at 4 h after injection resulting in 250 % increase in photoacoustic signal compared to the signal acquired from mice injected with unconjugated PEGylated gold nanostars. Moreover, tumor growth inhibition was also reported with an extension of survivability of tumor bearing mice.
Furthermore, introduction of gold nanospheres into a biological environment leads to complex interactions, such as aggregation of nanoparticles within cells, which can be visualized due to the resulting change in optical absorption spectra. Mallidi et al. [61] reported the synthesis and application of bioconjugated gold nanospheres (20 nm dia.) that can specifically bind to the cancer biomarker endothelial growth factor receptor (EGFR) for improved differentiation of cancer from background healthy tissue. EGFR-expressing human epithelial carcinoma cells (A431, n = 6) and EGFR-negative human breast cancer cells (MDA-MB-435, n = 3) were subcutaneously inoculated in mice followed by intravenous injection of EGFR-targeted or PEGylated gold nanospheres . Results of EGFR-targeted gold nanoparticles in A431 tumors are shown in Fig. 3a, b. A wavelength shift in the absorption of targeted gold nanospheres in EGFR expressing tumors was observed from 520 nm (green) to 720 nm (near infrared) due to plasmon resonance coupling. Effectively, cellular internalization and aggregation of nanoparticles into lysosomal compartments brings nanoparticle , and their individual free electron clouds, into close proximity. This grouping of nanoparticles then acts as a larger nanoparticle of a different shape with one associative electron cloud that has a distinct resonance frequency and associated optical absorption, changing the overall absorption spectrum from that of individual nanoparticles. Accumulation was confirmed by silver staining (which detects the presence of noble metals by depositing ionic silver until the nanoparticle can be visualized with light microscopy) of histological sections, along with an increase in photoacoustic signal over time (4 h after injection) shown in Fig. 3c, d. In contrast, images of mice with PEGylated gold nanospheres showed no significant change in photoacoustic signals, indicating that using targeted nanoparticles increased specificity in imaging EGFR -expressing cancer cells.
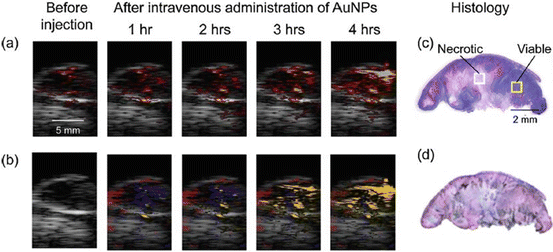
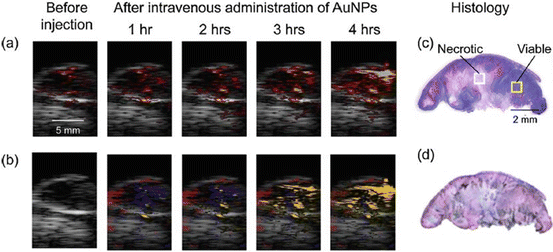
Fig. 3
Gold nanoparticles for photoacoustic imaging of cancer . (a) Photoacoustic and (b) multiwavelength spectroscopic photoacoustic images pseudo-colored to show oxygenated- (red) and deoxygenated hemoglobin (blue), and accumulation of gold nanoparticles (yellow) before and 1, 2, 3, and 4 h after intravenous injection of EGFR-targeted gold nanoparticles in a subcutaneous A431 breast cancer tumor. (c) H&E and (d) silver enhancement staining of histology tumor slices showing accumulation of EGFR-targeted gold nanoparticles within the tumor. Reprinted with permission from [61].
Overall, gold nanoparticles have been extensively used as contrast agents for photoacoustic imaging due to their excellent optical absorption and finely tunable absorption characteristic that can be optimized for specific applications. However, the bioaccumulation of gold nanoparticles remains a limiting factor in their use clinically.
3.5 Silver Nanoparticles
Silver nanoparticles have been commonly used in biomedical and cosmetic purposes for their antibacterial properties [62]. Unlike gold nanoparticles, silver nanoparticles dissolve over time in biological environments making them more suitable for repeat administrations for clinical purposes compared to gold nanoparticles [62]. However, the ability of silver nanoparticles to degrade introduces new challenges for their biomedical use, including potential silver toxicity [63] through the introduction of reactive oxygen species leading to cellular oxidative stress and lipid peroxidation [64] and decreased stability which would affect their desired optical absorption characteristics.
3.5.1 Synthesis
Silver nanoparticles are synthesized very similarly to gold nanoparticles, predominantly through seed-mediated growth procedures. Reducing agents appropriate for silver particles include hydrazine, ascorbic acid, temperature increases, and light exposure. Silver seeds approximately 3 nm in diameter can be synthesized by the reduction of silver nitrate (AgNO3) using sodium citrate and adding sodium borohydride (NaBH4) in a dropwise fashion, followed by stabilization by bis(p-sulfonatophenyl)phenylphosphine dihydrate dipotassium (BSPP) [65]. The further growth on anisotropic shapes is accomplished similarly to that of gold nanoparticles through the addition of silver salts and reducing agents [66].
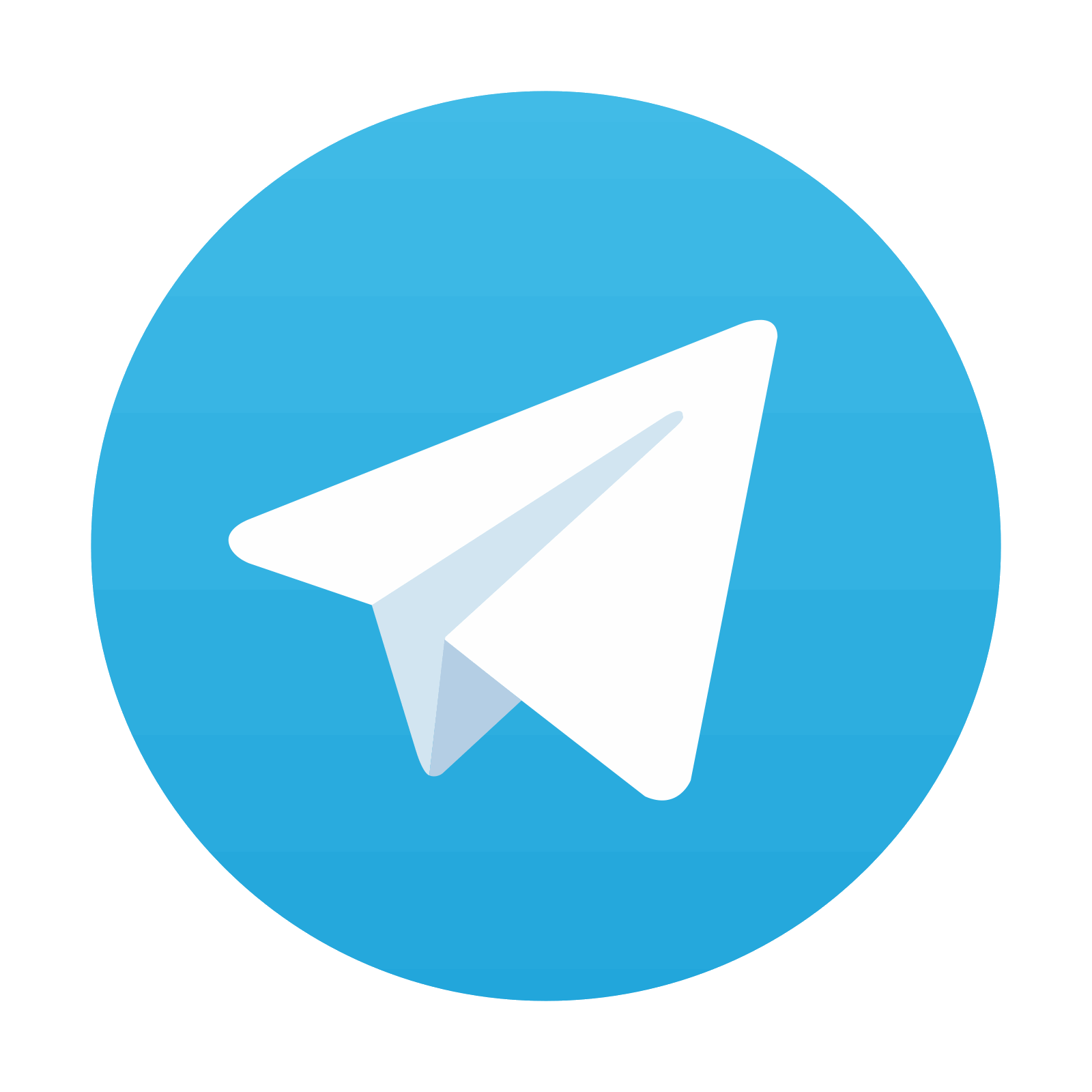
Stay updated, free articles. Join our Telegram channel
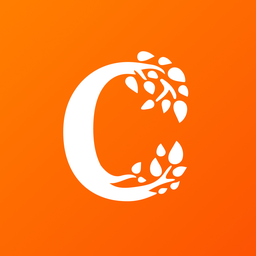
Full access? Get Clinical Tree
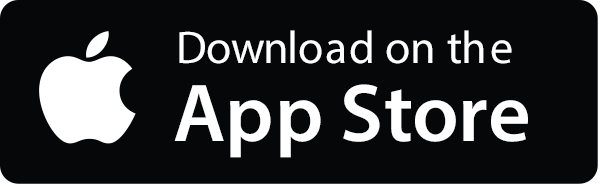
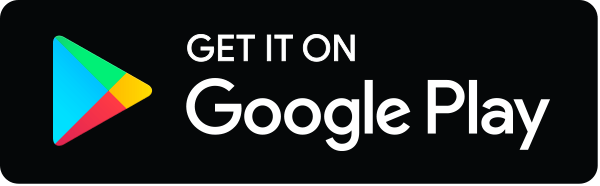