Fig. 14.1
(a) Microarray of gene expression. (b) Tissue library of human brain tumors with IHC staining for Olig2. Courtesy of Santosh Kesari, Brigham and Women’s Hospital. Current address is University of California San Diego School of Medicine
Some of the key prerequisites for imaging biochemical processes in living systems include adequate substrate (mRNA, proteins, surface receptors, etc.) to serve as molecular targets, the development of high affinity probes to bind to the targets, and the availability of sensitive and high resolution imaging modalities. Typically, for a successful imaging system, all of these prerequisites are met.
This chapter explores recent advances that have been made in molecular imaging with discussion of their potential diagnostic and therapeutic applications in the clinic. We begin by discussing key targets and the limitations of current modalities in molecular imaging studies. We then highlight the versatility of nanoparticles and cellular probes and how this has significantly advanced our ability to study molecular events in vivo.
Molecular Targets
The question of identifying where to begin interrogating cellular processes using molecular imaging starts with the central dogma of molecular biology. The dogma provides a framework for understanding the flow of sequence information beginning with deoxyribonucleic acid (DNA) and ending with protein products that affect cellular physiology (Fig. 14.2). DNA is first transcribed to form messenger ribonucleic acid (mRNA) in the cell nucleus. The mRNA is then processed and transported into the cytoplasm where it is translated using the cell ribosome machinery to form proteins, which undergo further posttranslational modification to their fully functioning form.
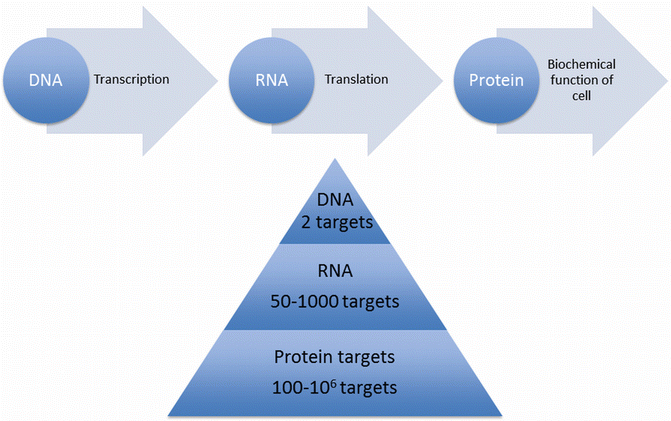
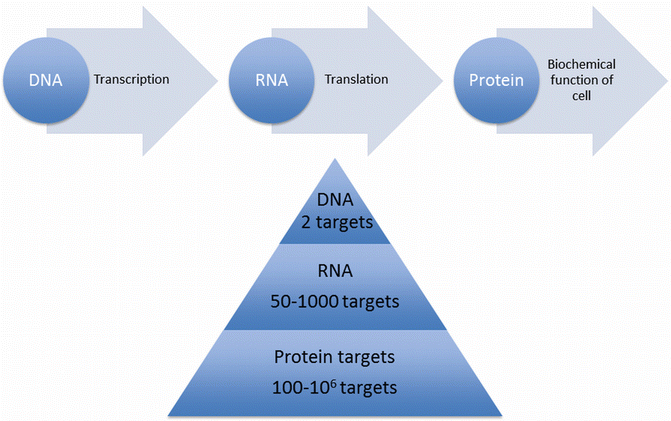
Fig. 14.2
Central dogma of molecular biology: DNA is transcribed into RNA, which is then translated to proteins. The number of targets increases several fold at each step, with any given cell containing two genes of DNA, between 50 and 1,000 mRNA molecules, and up to 106 protein molecules for each target
The regulation points of each of these processes are key targets for molecular imaging techniques. The number of genetic targets increases exponentially down the transcription pathway, beginning with two genes of DNA, to 50–1,000 mRNA and 100–106 protein polypeptides, whose function further amplifies the number of targets several fold [4]. The Human Genome Project has opened an exciting array of new opportunities by uncovering the entire collection of over 20,000 human genes, and therefore the entire spectrum of possible molecular targets.
The search and design of potential high-affinity probes has been complemented by a number of rapidly evolving technologies and discoveries such as the development of combinatorial techniques, rational design studies, high-throughput testing, robotics, and identification of molecular targets [4]. The important challenges to successful probe design include delivery barriers for intracellular targets, since high molecular weight macromolecules do not diffuse well within the cytosol, nor do they enter the cell easily. DNA is replicated, transcribed, and stored in the cell nucleus, thus making it a particularly difficult target. To reach a DNA target, a molecular probe must traverse the cell membrane (to enter the cytoplasm) and then traverse the nuclear envelope, also a lipid bilayer. Intracellular proteins and mRNA are more accessible as they are stored and processed in the cytoplasm, therefore requiring traversal of only one membrane barrier. Cell-surface proteins are the most accessible targets to molecular probes, as binding does not require the probe to cross cell membranes (Fig. 14.3). Molecular probes may utilize a diversity of available membrane transporters and channels to transverse biological membranes. These proteins may be influenced by ligand binding, voltage sensitivity or second messenger systems. They include channels that allow for the passive diffusion of specific molecules, uniporters that facilitate the transport of large molecules down their concentration gradient, and symporters and antiporters. Symporters couple transport of one molecule with another molecule present in the cell environment, down its concentration gradient, and antiporters transport molecules actively, against their gradient, along with a molecule that has a favorable gradient. Other challenges include ensuring a sufficient concentration of probe is bound to its molecular target for enough time to be detectable in vivo. Conversely, unbound probe should not be present in appreciable quantities as this would result in a nonspecific “background” signal.
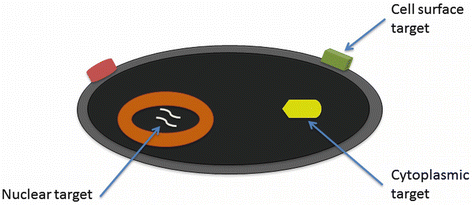
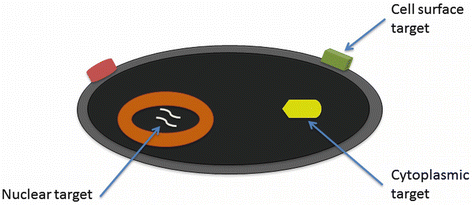
Fig. 14.3
Accessibility of molecular targets in a cell. Cell surface targets, such as surface proteins, are the most accessible since probes do not have to cross membrane barriers. Cytoplasmic targets, which include most intracellular proteins and mRNA, require a probe to traverse the plasma membrane. Nuclear targets, such as DNA, are the least accessible since probes must traverse both plasma and nuclear membranes
While significant effort has been made to design more efficient methods for the identification and delivery of probes, a number of procedures have been used in drug delivery to bypass the physiological barriers that exist to prevent foreign substances from circulating within the body. Such procedures include the use of drugs that circulate for longer periods of time to obtain a more homogeneous distribution, thus allowing the probe to distribute to all accessible regions of the cell. Other procedures include the local delivery of probes in conjunction with pharmacological methods to improve targeting and pegylation of the drug to decrease its identification by the immune system, thus increasing the amount of time the probe remains in circulation [4, 5]. In addition, the use of signals, derived from peptides, which stimulate shuttling of the drug across cellular membranes has also been used [6]. This method directly uses the ability of the cell to shuttle molecules into the intracellular space using ligand activated transporters on the surface of the cell membrane.
DNA and mRNA are present at relatively low concentrations in cells and therefore would require levels of signal amplification when used as targets in MI that are difficult to achieve in clinical imaging [4]. In contrast, imaging of protein targets is efficient and practical, and a number of biochemical strategies have been developed for amplification of protein signals. These strategies have utilized the alteration of the physical and chemical behavior of probes after target interactions, capitalizing on unique cellular functions to trap probes, and pre-targeting and enhanced probe kinetics to increase target concentrations [4, 7–10].
Three classifications of molecular probes are generally discussed and utilized in MI modalities [11]. Compartmental probes are generally used to assess physiological parameters such as flow and perfusion (Fig. 14.4). Strictly speaking, it is not the molecular process being studied but a related biochemical interaction. Targeted probes are designed to complement a specific moiety targeted to the molecule, enzyme, or receptor of interest and include a component that provides physical contrast [11] (Fig. 14.5). The enhancement of angiogenic vessels in rats has been demonstrated in the magnetic resonance modality using antibodies against αvβ3 integrin, a transmembrane protein characteristic of angiogenic endothelium. These antibodies are bound to liposomes containing several Gd(III) moieties, which significantly increased relaxivity of the probe [12]. Finally, smart probes activate exclusively in the presence of a specific molecular agent [11]. These probes hold an advantage over targeted probes due to the absence of significant background signal. Perhaps the best known example of a smart probe is EgadMe, which consists of a Gd(III) chelating agent bound at eight coordination sites and a galactopyranose residue that blocks the remaining site. In the presences of β-galactosidase, the galactopyranose is cleaved therefore allowing water to access the blocked Gd(III) coordination site [13]. Other examples of smart probes include zinc and calcium activated gadolinium magnetic resonance contrast agents, which have demonstrated potential use in signal transduction and catalytic activity studies [14, 15]. Recent advances in probe technology have included the development of nanosensors that are able to detect specific DNA and RNA nucleotide pairings, in addition to hybridization chain reaction (HCR) and RNA fluorescence in-situ hybridization (FISH) technologies for detecting individual RNA molecules [16–18].
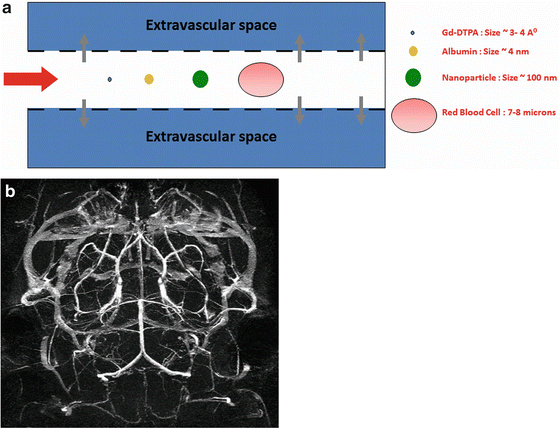
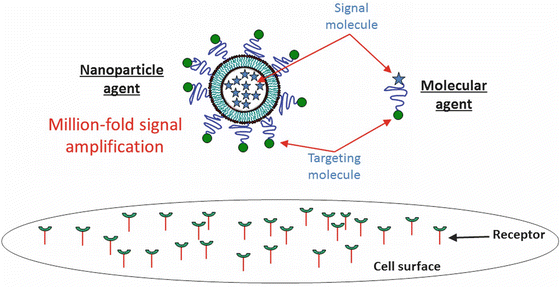
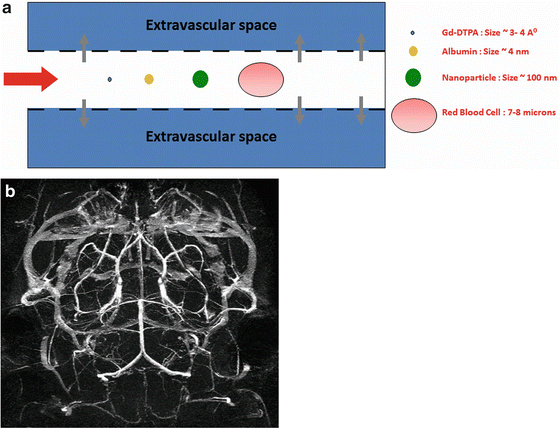
Fig. 14.4
(a) Illustration of a compartmental probe. Conventional contrast agents are sub-nm in size and can leak into the extravascular space. Nanoparticles, being typically 20–50-fold larger than traditional contrast agents, remain in the intravascular space. (b) MR Angiography demonstrating the mouse Circle of Willis obtained using a surface-conjugated liposomal gadolinium contrast agent, a vascular compartmental probe
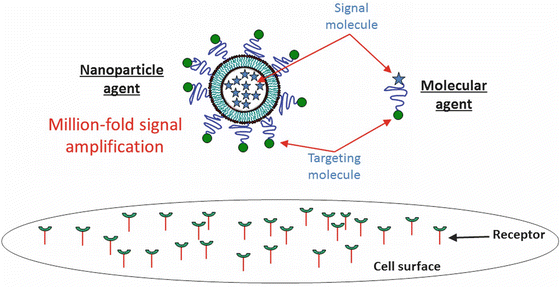
Fig. 14.5
Illustration of nanoparticle and conventional molecular targeted agents. A nanoparticle agent can enable a million-fold signal amplification by encapsulating millions of signaling molecules
Imaging Modalities
Prior to approaching any biological question, it is also essential to consider the most suitable imaging modality for the problem, in addition to identifying a suitable target. The conventional wisdom for molecular imaging modalities has been limited to nanosensor, optical, nuclear, and, to a lesser extent, MR imaging, with limited applications of the commonly used CT and ultra sound (US) modalities [4]. Recent advances in imaging technologies have seen broader molecular imaging applications of all of these and have resulted in variations such as photoacoustic ultrasound, fluorescence or luminescence imaging, and MR microscopy [11, 19]. Current MI modalities available include CT, MR, positron emission tomography (PET), single photon emission CT (SPECT), optical imaging techniques, and US [19]. These modalities differ in their sensitivity, spatial and temporal resolution, and complexity of use; however, they are applied with a shared goal of higher specificity. The important properties of several common MI modalities will be discussed in the following paragraphs.
Nuclear Imaging
Nuclear imaging has been used extensively for molecular imaging at the organ level, with recent extensions to the tissue, cellular, and genetic level. The fundamental concept underlying this imaging modality is that proteins in the cell can be probed with specific radiopharmaceuticals. The two classes of marker genes that have been largely investigated are those that encode cell-surface proteins and those that encode intracellular enzymes [4]. The two prominent nuclear imaging techniques currently in use are PET and SPECT.
PET has been used for molecular, physiologic and, to a lesser degree, anatomic imaging, and more specifically, has been used extensively for metabolism and in the preclinical realm, gene expression studies. It remains the paradigm for sensitivity to molecular events with nanomolar to femtomolar (10−9–10−15) sensitivity for biological compounds [11]. Its sensitivity provides the opportunity to quantitatively characterize receptors and ligands at molecular concentrations. PET technologies have resolutions of around 3 mm. Some commonly used radionuclides, with their corresponding half-lives, include 15O (2.07 min), 13N (10 min), 11C (20.3 min), 18F (1.83 h), 124I (4.2 days), and 94Tm (53 min) [11]. The disadvantages of this modality include the cost and accessibility of hardware, and availability of radionuclides. Gene expression studies using this modality have been conducted by incorporating a PET reporter gene, such as that for the intracellular enzyme herpes simplex virus thymidine kinase, into an adenovirus vector that is able to penetrate the cell nucleus. The final intracellular protein product of the reporter gene after transcription and translation can be detected by treatment of the cells with 8-[F-18]fluoroganciclovir, which penetrates the cell membrane and binds to the protein. Analogous studies have been conducted by utilizing reporter genes that produce cell surface receptor targets, such as the dopamine receptor, which are bound by [F-18]flurorethylspiperone [20].
SPECT is another powerful nuclear imaging technique that has been used largely for gene expression and structure studies. It provides a cheaper alternative to PET at the cost of much lower resolution, often around 1–2 cm. Some commonly used radionuclides for SPECT include 99Tcm (6 h), 111In (2.8 days), 123I (13.2 h) and 125I (59.5 days) [11]. Disadvantages of this technique are the limited availability of radionuclides and complicated probe chemistry, since, unlike PET, it does not use physiological tracers and therefore requires chelating agents to incorporate the radionuclide within the tracer [19]. Radiolabeled Annexin V, which binds phosphatidylserine, a cell surface protein expressed during programmed cell death, has demonstrated the use of SPECT in detection of apoptosis and protein targets [19, 21]. Furthermore, radiolabeling of DNA oligonucleotides with 99Tcm has made mRNA and DNA targets accessible to SPECT studies [22, 23].
Optical and Bioluminescence Imaging
In recent years, optical imaging technologies have had a significant impact on the landscape of molecular imaging. These technologies have advanced from a better understanding of fluorescence, which is the absorption of one wavelength of light and its subsequent emission, and luminescence, which arises from the conversion of chemical energy into light [11]. While optical imaging technologies do not have any current clinical applications, they are much cheaper compared to the hardware requirements of other modalities and have been used for noninvasive anatomical localization. General optical imaging techniques have resolutions that range from 3 to 5 mm and possess nanomolar sensitivity. Some of the major disadvantages of general optical imaging techniques include low resolution and low depth penetration.
Fluorescence in the near infrared region is best of the optical imaging techniques and may be used to obtain greatest depth since the lowest coefficient of absorbance occurs in the 650–900 nm range, which coincides with minimal inherent fluorescence of body tissue [4, 11, 19]. This mode of optical imaging requires a light source of a defined bandwidth, which upon contact with an optical contrast agent, or fluorescent molecule, emits a signal with different spectral characteristics. This signal can then be resolved with an emission filter and captured with high sensitivity [3, 4]. Optical contrast agents that are detectable in the near infrared range have been designed by conjugating analogs of somatostatin, which bind to somatostatin cell surface receptors overexpressed in tumors, to cyanine dye [24]. In addition, near infrared labeled probes have been used to image protease activity of intracellular enzymes such as cathepsin B [10].
Bioluminescent imaging involves the administration of a substrate, luciferin, which emits light at specific wavelengths upon reaction with an enzyme, luciferase. Since most animals have no endogenous luciferase enzyme, there is very little background noise from this technique. This modality has low resolution, on the range of 3–5 mm, and is used largely for anatomical localization, gene expression and functional studies, and high-throughput screening [11, 19, 25]. Reporter genes encoding the firefly photoprotein luciferase, which utilizes energy from ATP to emit photons, have been used to image the distribution and clearance kinetics of tumor cells [26].
Magnetic Resonance Imaging
Magnetic Resonance (MR) Imaging has been used extensively in the clinic and is capable of extracting anatomical and physiological information concurrently. It is used primarily for anatomical evaluation and exhibits a resolution on the range of 1–2 mm. MR techniques are not naturally sensitive to label detection, and thus have low signal yield. As a result, they require robust signal amplification strategies such as cellular internalization of paramagnetic probes, contrast agents coupled with biological amplification strategies, such as trapping of probes within a cell, or secondary reporter systems, such as the use of an enzyme to catalyze the formation of detectable molecules in cells [27
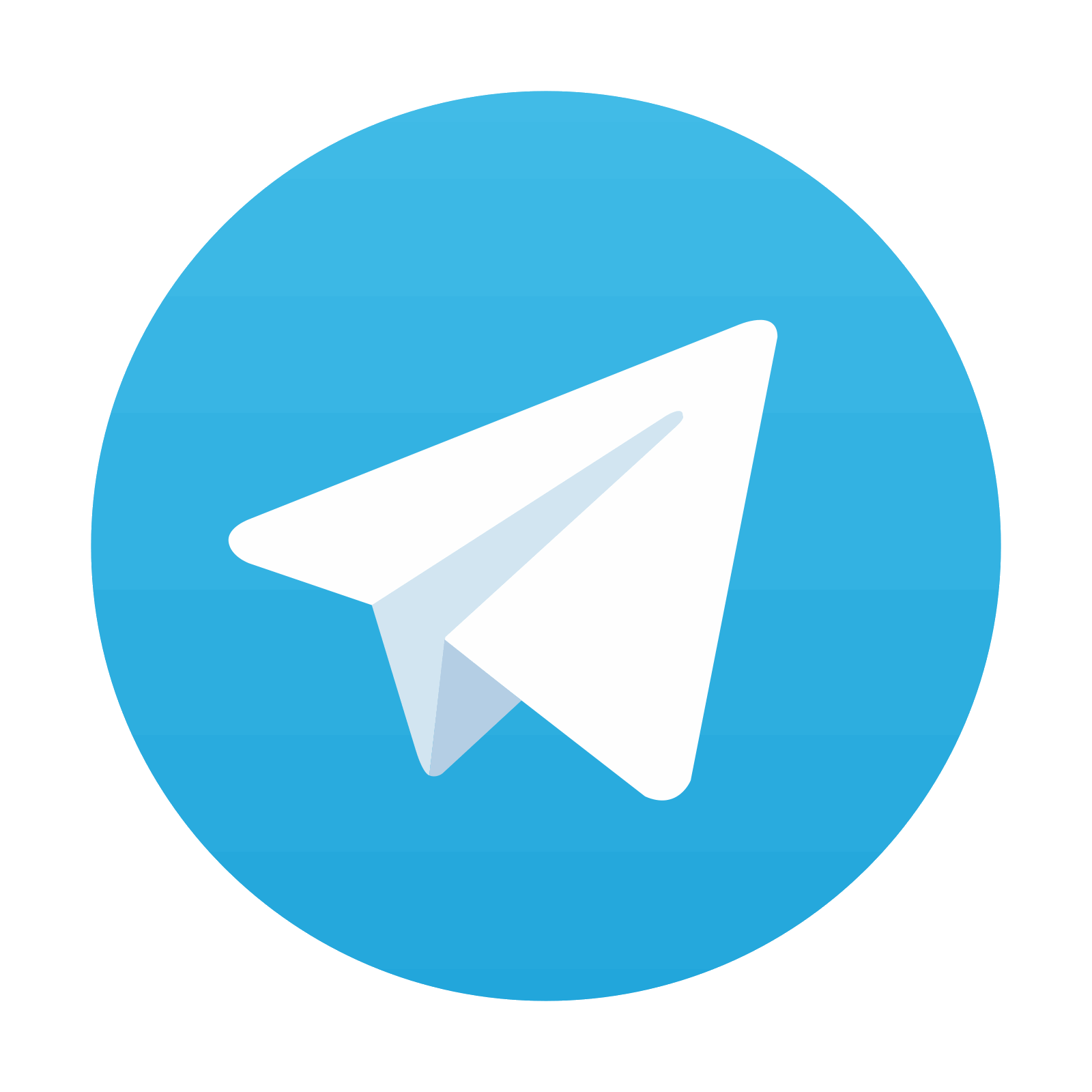
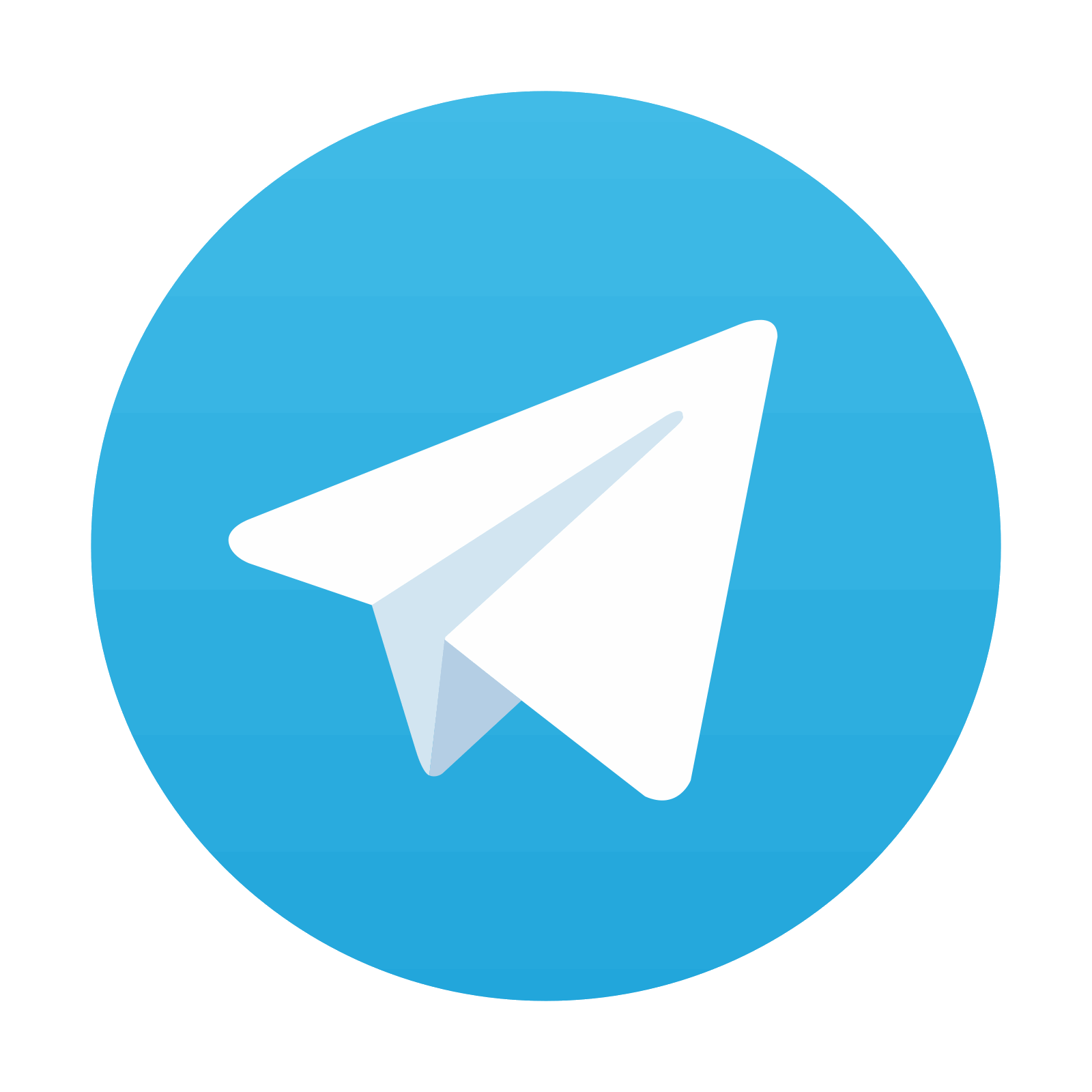
Stay updated, free articles. Join our Telegram channel
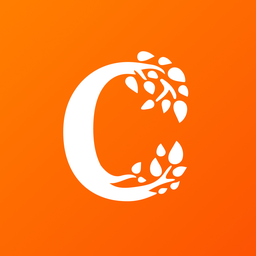
Full access? Get Clinical Tree
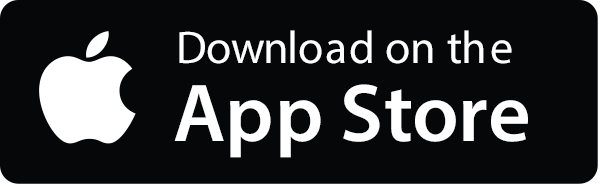
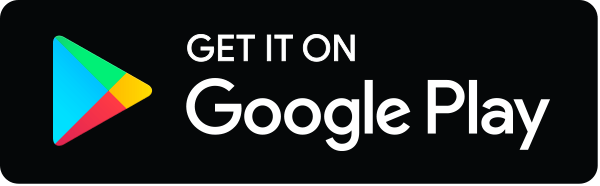