is the ratio of induced magnetization to the source field and μ 0 is the magnetic permeability in vacuum.
Although the distinction between source and induced magnetic field tends to be neglected in practice, in MR diagnostic imaging, it has an important role when the susceptibility has an appreciable positive value, as is the case of paramagnetic contrast agents and ferromagnetic objects.
When an object, such as the human body, a metal prosthesis, or an erythrocyte, is introduced into a MR tomograph, it is subjected to translation forces in the gradient zones and to rotation forces with respect to the direction of the static field B. Such forces depend on the susceptibility of the material from which the object is made, on its shape and volume, and on the intensity of the magnetic field, and in relation to them, they may be negligible or have potentially lethal effects.
The force of translation
depends on the volume V, the susceptibility value, and the magnetic field gradient, whereas the force of rotation required to oppose the object’s rotation
also depends on its length L and volume, thus on its shape.
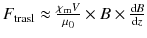
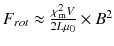
In general, F rot >> F trasl, thus the tissue damage induced by the rotation force generated by the field of an implanted metal object will be greater than the damage caused by the force of translation, especially in the presence of high magnetic fields, due to its quadratic dependence on B [6].
In human tissue, the magneto-mechanical effects are negligible due to their weak susceptibility; for instance, the alignment of erythrocytes parallel to the magnetic field due to their ellipsoid shape is negligible because of the quadratic dependence of the torque on susceptibility. The force acting on 100 g of water in an 8 T magnetic field with a peak gradient of 7.9 T/m and a product
, equaling 43 T2/m, is negative and is ca. −3.4·10−3 N.

By contrast, a 100 g stainless steel wrench with susceptibility of 0.01 and a volume of 12.5 cm3 will be attracted to the same field with a force of 4.3 N, an acceleration of 43 m/s2, that is, more than four times of the gravitation, turning it into a bullet [3, 6]. The magnitude of the force acquired for the paramagnetic and ferromagnetic objects would be larger by a factor 102 to 105, posing serious hazards for workers and patients in the magnet area.
People with splinters lodged in the bodies or wearing metal prostheses are also at high risk, since these object’s movements, induced by the magnetic field, may cause tissue or vessel tears.
Inside the magnet, the field gradient is zero and so is the force of translation exerted on a ferromagnetic object, which will thus remain trapped in the tunnel. Attempts to extract it forcibly in case of an accident are often vain and can impair the magnet’s stability in the cryostat. Recovery of the object usually requires inactivation of the magnet. By contrast, the rotation forces, which depend solely on B 2, continue to be active. To prevent this type of risk, metal objects must never be introduced into the scan room. This is clearly stated in notices and is prevented from happening by taking an accurate patient history.
Since most of the data on the compatibility of several implanted devices and prostheses regard 1.5 T static magnetic fields, MR investigations of such patients at higher fields are to be avoided.
However, information on the safety and compatibility of 26 aortic aneurysm clips tested at 8.0 T and of 109 implants and devices tested at 3.0 T has been published.
Safety in this connection indicates that the objects have been shown to carry no additional patient risk save a reduction in image quality, whereas compatibility indicates demonstration of the property of not interference with image quality. The distinction between long- and short-bore magnets of equal field intensity lies in the differences in the respective position and height of gradient peaks at the tunnel aperture [7–10].
In general, patients wearing pacemakers should avoid undergoing MR imaging, especially at high magnetic field. The several effects that may derive from exposure are due to the static magnetic field (e.g., pacemaker movement, remote control switch-off, and ECG changes), to the RF field (e.g., heating, alteration of pace frequency, and device reset), and to the gradients (e.g., induction of voltage, heating, and remote control switch-off). However, these effects still present controversial aspects because, on the one hand, early compatibility studies assessed models that are no longer in use and, on the other, recent data indicate that small groups of patients underwent MR imaging for vital reasons without experiencing adverse effects [11, 12, 14–18].
In most materials, the magnetization induced is parallel to H; in such cases, M, B, and H all point in the same direction, and the material is said to have isotropic susceptibility. If, however, the susceptibility varies within the volume of the material exposed to the magnetic field, the object becomes magnetized along directions that may not be parallel to the magnetic field; in anisotropic cell structures, this may lead to structure reorientation or distortion. In such cases, this effect, which has been documented in vitro, is stronger than the one induced by object shape [6].
Another biological effect connected with a potential risk, which in the past has been attributed to abnormal myocardial repolarization, is in fact induced by the blood flow in the large vessels within a strong magnetic field. The Lorentz force, F, induces on vessel walls an electric field
, where v is blood velocity, θ the angle between the flow direction and the direction of the magnetic field, and q the charge of the ions in the blood.
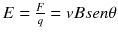
This induced electric field causes a reversible increment in T-wave amplitude in the ECG. In vivo investigation of this effect at fields greater than 8 T has demonstrated that the induced potentials are below the threshold of nerve or muscle stimulation. The migration of opposite charges to vessel walls induced by the magnetic field also causes a current in a direction perpendicular to the flow velocity in vessels and thus an additional Lorentz force in a direction opposite to the blood flow that could contrast it appreciably. However, it has been demonstrated that this effect is negligible even in the presence of magnetic fields exceeding 10 T [13].
2.2 Varying Electric and Magnetic Fields
During scanning, patients are also exposed to the activation of magnetic field ramps or gradients for spatial localization of the signal and to RF impulses for signal generation and decoding. Specific effects that may constitute a source of patient risk during scanning have been documented in their connection.
2.2.1 Magnetic Field Gradients
Many of the effects ascribed to magnetic fields that vary over time are in fact related to the associated electric field. Switching the magnetic field gradients on and off may induce electric currents capable of affecting the cell membrane potential and, if sufficiently intense, of stimulating the peripheral nervous system and the cardiac muscle. The stimulation threshold of peripheral nerves may be painful but is reversible and is used as a safety reference indicator for cardiac stimulation, which in contrast carries a risk of fibrillation. In fact, with ramp durations of less than 1 m/s, the former threshold is always lower than, and is reached before, the latter [13].
The intensity of these currents is proportional to the induced electric field and depends on the conductivity of biological tissues. The other critical factor of gradients, besides their amplitude, is their slew rate; the value of the induced electric field can be calculated using a model represented by a cylindrical object with radius r as follows:
.

For instance, if during an examination a localization ramp from 0 to 20 mT/m in 200 μs is launched from a 1 m coil, then the temporal variation of the magnetic field will be 100 T/s, and for an abdomen 0.4 m in diameter, the value of the electric field calculated using the above model will be 10 V/m, which exceeds the 6 V/m threshold of peripheral nerve stimulation [4, 20].
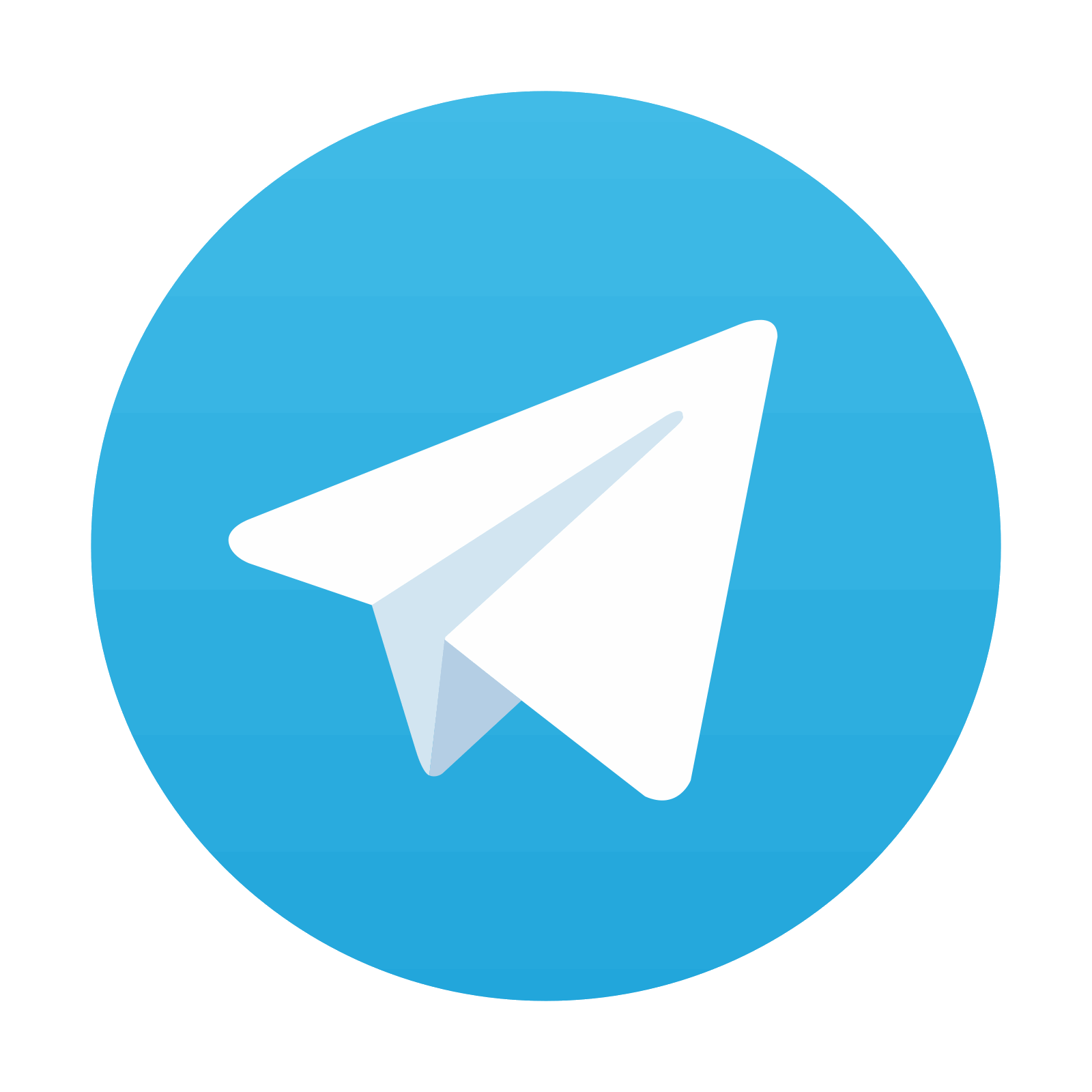
Stay updated, free articles. Join our Telegram channel
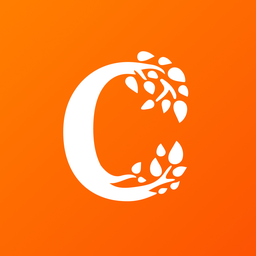
Full access? Get Clinical Tree
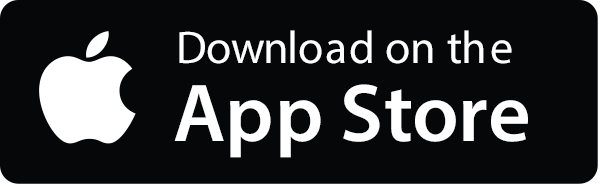
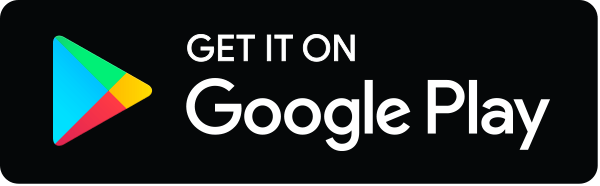