Fig. 11.1
The prefrontal cortex division based on Brodmann areas (Modified from Petrites and Pandaya (1994))
11.2.2 Learning from Lesion Studies
The functional impairments derived from lesions have been one of the first forms to access the functional neuroanatomy of the PFC. Multiple studies evaluated the consequences of surgical lesions in animals. Obviously the readout restrictions of an animal behavior compose the main limitation and interpretation pitfall. Even when studying nonhuman primates, presenting more similar cognitive skills to humans, this limitation is reduced but still present. Therefore, studies of human PFC spontaneous lesions became so valuable (Milner 1982; Leimkuhler and Mesulam 1985; Owen et al. 1990; Bechara et al. 2000; Stuss et al. 2000, 2001; Fellows and Farah 2005a). A more recent publication with a vast sample of patients systematically addressing cognitive impairments (Gläscher et al. 2012) re-incited the discussion about the specificity of cognitive correlates (Fig. 11.2). The color overlays represent affected regions, each color associated with reduced performance in a specific task. Here, the PFC correlates related to different cognitive functions such as cognitive control, conflict monitoring, response inhibition, flexibility, planning, working memory (functions detailed over the next section), and decision-making supported the segregation of two distinct groups. The first involved the DLPFC and VLPFC in association with cognitive control, namely, conflict monitoring, response inhibition, and switching/flexibility. The second comprised the medial/anterior PFC and the OFC and was associated to value-based decision-making in a gambling task, which requires assessment of internal information such as individual preferences. Lesions in the rostral ACC could not be classified in one or another of these groups. Also, the dorsal ACC has not been specifically related to Stroop impairment corroborating to previous findings (Fellows and Farah 2005b). This reinforces a certain degree of participation across tasks in these ACC regions, instead of showing clear-cut task divisions. After all, integration should be advantageous for the optimization of resources regarding the multiplicity of stimuli repeatedly perceived by our sensory system. Nevertheless, one should always consider that lesion-deficit studies can give valuable insights about the necessity of certain region(s) during behavioral performance, but will rarely allow more specific inferences about the functional significance of this region(s) in the network. Therefore, functional studies are imperative to further elucidate the neural correlates of cognition.
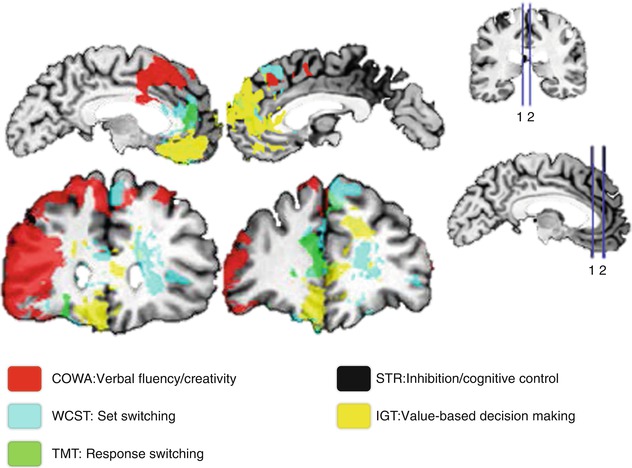
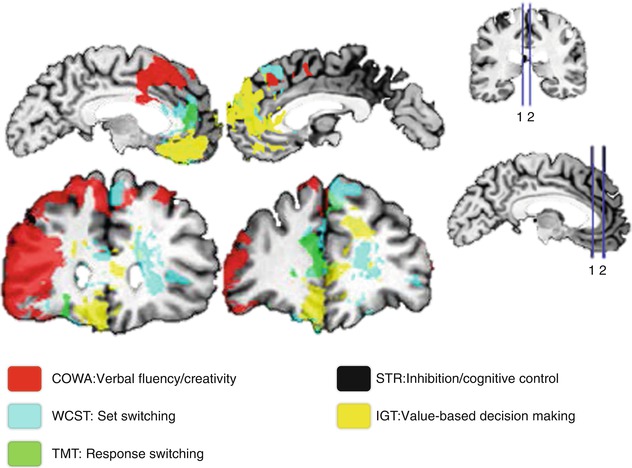
Fig. 11.2
Spatial lesion mapping associated with cognitive impairments. 1, 2 spatially represent the sections presented in the left side of the figure (Modified from Gläscher et al. (2012))
11.3 Imaging Cognitive Functions
We are daily challenged to respond and adapt to the environment around us. The capacity to evaluate and decide over multiple alternatives is essential for optimizing resources and surviving. Hence, it is demanded from the brain to be functionally a dynamic organ, in which components interact and solutions are achieved by a product of perception and experience. Laboratory functional imaging studies have been used toward further understanding of the complex construct of cognition.
11.3.1 Executive Functions
There has been so far little consensus on the taxonomy of attention and executive control functions. Uncertainties about the classification of such functions derive at least partially from the overlapping correlates behind the psychological constructs of selective attention, response inhibition, cognitive control, and working memory. For instance, numerous neurophysiological and functional imaging studies have collected evidence for very similar prefrontoparietal and prefrontotemporal neural correlates involved in both short-term maintenance of task-relevant information in working memory and selective attention processes (Gruber and Goschke 2004; Ikkai and Curtis 2011; Gazzaley and Nobre 2012; Niendam et al. 2012). Selective attention, the ability of focusing on specific relevant information over the background, is gained through sensory upregulation guided by the PFC (Hopfinger et al. 2000; Kastner and Ungerleider 2000; Petersen and Posner 2012). The sensory areas processing irrelevant and possibly interfering information will be downregulated. At this point, a close relationship between the psychological constructs of attention, executive, and cognitive control can be observed. Such modulatory processes can interfere on sensory perception and processing of a certain object or aspect from the environment. These are top-down-oriented processes that control bottom-up-oriented attention processes (Fig. 11.3). The first can allow us to succeed daily in different cognitive tasks; the second can alert us from any threat unexpectedly arriving that may endanger our integrity. A frequent example is the one about a student preparing for an exam (top-down), while the fire alarm goes off (bottom-up). These two forces compete for the attention resources as shown (Corbetta and Shulman 2002; Gruber and Goschke 2004; Posner 2012; Petersen and Posner 2012). In the laboratory, the bottom-up component of attention can be investigated with a task called oddball paradigm (Bledowski et al. 2004; Melcher and Gruber 2006; Gruber et al. 2009). Here, an infrequent stimulus is presented among other frequent stimuli. The fact that it is rarer triggers attention, a process called stimulus-driven attention (Corbetta and Shulman 2002).
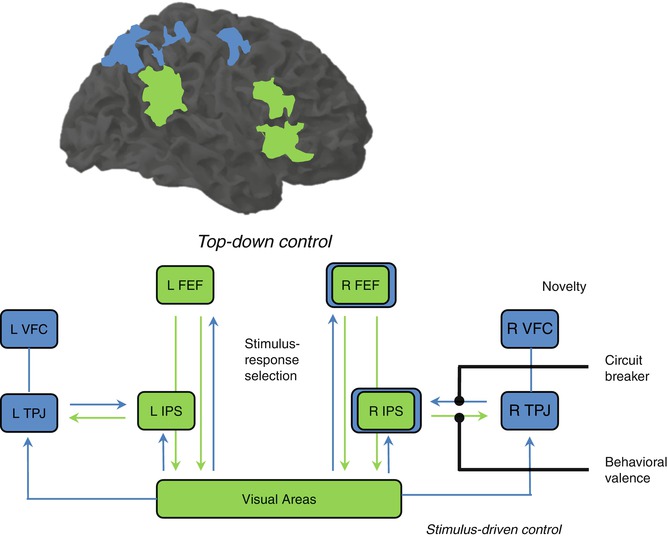
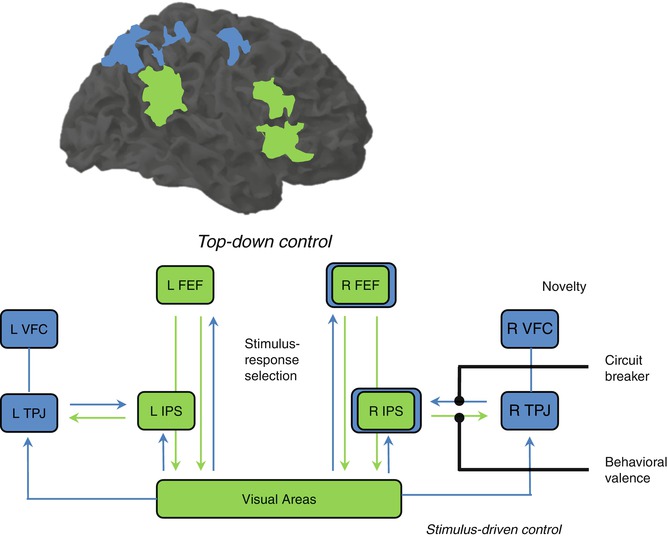
Fig. 11.3
Dorsal and ventral frontoparietal areas involved in top-down and bottom-up attention processes (Modified from Corbetta and Shulman (2002)). FEF frontal eye field, IPS intraparietal sulcus, TPJ temporoparietal junction, VFC ventral frontal cortex
A paradigm called Go-Nogo demands response inhibition of infrequent stimuli. Subjects are instructed to respond to frequent stimuli in such a regular and fast fashion that a tendency to respond is developed, making it difficult to avoid responding even when a Nogo infrequent stimulus is presented. The inferior frontal gyrus has been implied in the capacity to suspend this initiated response as part of a supramodal network (Chikazoe et al. 2007; Walther et al. 2010). Also, the activation in this region during inhibition has been correlated with the variability in impulsivity traits measured from a behavioral scale (Goya-Maldonado et al. 2010). These findings suggest that the IFG, as an area involved in cognitive control, regulates the expression of inherent impulsive tendencies in healthy subjects. Most interestingly, the same area has been reported to be involved in both response inhibition and stimulus-driven attention processes (Fig 11.4).
Another laboratory approach of inhibition called Stroop paradigm deals with conflict detection and cognitive control. While responding to the color of letters displayed, one reads from the letters, another color name leading to conflict. Response conflict and semantic conflict were identified in Stroop paradigm (Melcher and Gruber 2009), and there are now many variations of the paradigm (Stroop 1935, 1992; MacLeod 1991; Kerns et al. 2004; Matsumoto and Tanaka 2004; Melcher and Gruber 2006), but the principle remains that contradictory information is displayed and conflict has to be identified and solved to achieve the correct answer. With the final purpose of succeeding, adjustments based on additional information from the environment are demanded. This recruits the DLPFC for increased cognitive control, which is again deactivated once the conflict disappears (Fig. 11.5).
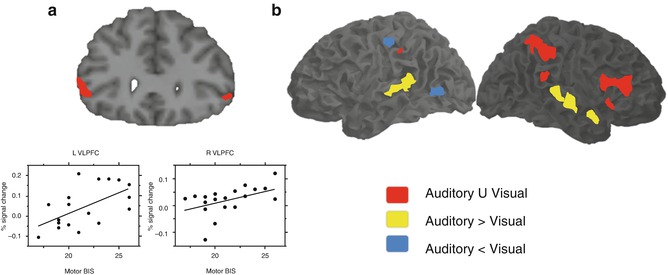
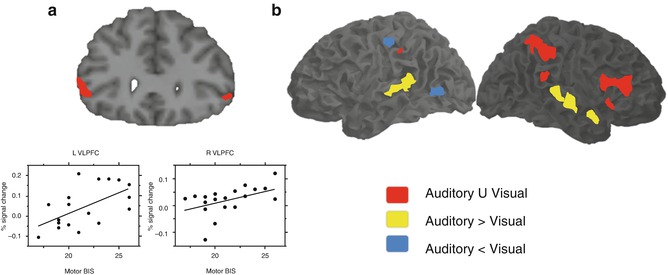
Fig. 11.4
Neural correlates of response inhibition elicited by Go-Nogo paradigm (a) which correlated to impulsivity scores, (b) presented supramodal (red areas) characteristics (Modified from Goya-Maldonado et al. (2010), Walther et al. (2010)). L VLPFC left ventrolateral prefrontal cortex, R VLPFC right ventrolateral prefrontal cortex, BIS Barratt Impulsiveness Scale
To address the dynamic mechanisms involved in bottom-up and top-down components of behavioral control, a more recent paradigm called the desire-reason-dilemma was used (Diekhof and Gruber 2010; Diekhof et al. 2011). It consists of two settings, one that allows the acceptance (desire) of previously conditioned rewards while the subject performs a simple task and another that restricts the acceptance (reason) of rewards. The nucleus accumbens and ventral tegmental area, regions involved in the dopaminergic bottom-up of the reward system, have shown high activation during desire, which was drastically top-down suppressed during reason by specific prefrontal regions.
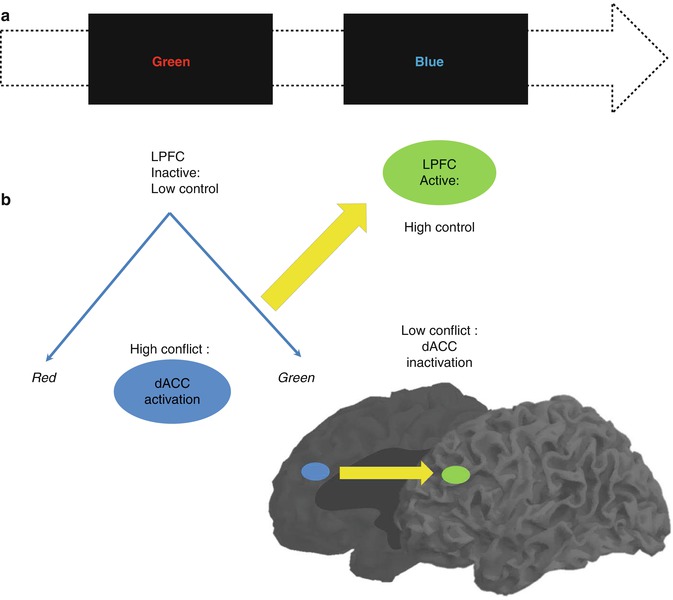
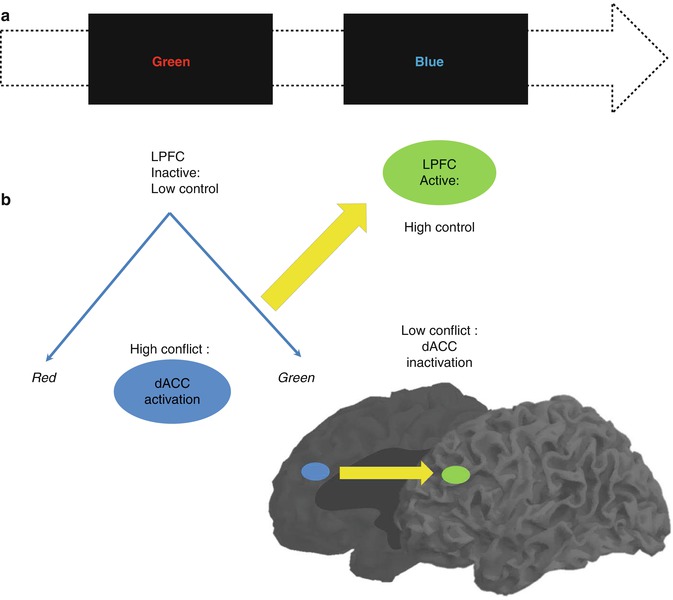
Fig. 11.5
(a) Color and names of colors presented as conflict stimuli in the Stroop paradigm and (b) the medial and lateral areas involved in conflict detection and cognitive control, respectively (Modified from Matsumoto and Tanaka (2004), Kerns et al. (2004)). LPFC lateral prefrontal cortex, dACC dorsal anterior cingulate cortex
11.3.2 Working Memory
Working memory or short-term memory is a cognitive function that allows holding an amount of information for a limited period of time so a certain goal can be achieved. The information can be captured from the environment and may be discarded right after it has served its purpose. This enables a faster and more effective performance of daily tasks. Analogous to the input of external information, parts of consolidated memory can be retrieved and put online during the performance of language, problem solving, and task planning.
Multiple cognitive models have been proposed, among which the model of Baddeley and Hitch (1974) was highly influential. Here and in a more actual version (Baddeley 2000), short-term memory processes could be partitioned in different components. Studies addressing the functional anatomy of working memory were then conducted in the early 1990s, based on this cognitive model (Baddeley and Hitch 1974). These components described as part of the working memory were suggested to be separable in PET and fMRI studies (Jonides et al.1993; Paulesu et al.1993; D’Esposito et al. 1995), and the verbal and visual components could be later compared (Fig. 11.6b). However, this segregation was not confirmed by following fMRI studies using the n-back paradigm (D’Esposito et al. 1998; Nystrom et al. 2000; Zurowski et al. 2002; Owen et al. 2005). This task involves responding with a button press every time a stimulus is repeated in the sequential presentation. The n represents the position in which this repetition should be tracked and is instructed right before the task block begins; for instance, 1-back means that a button press is demanded every time a stimulus is immediately repeated, 2-back every time a stimulus is repeated in an interval of another stimulus, 3-back every time it is repeated in an interval of two other stimuli, and so on. Clearly the level of difficulty increases with the n, so that the information has to be hold for longer and across more in-between stimuli. The use of this task in a psychiatric sample withholds the disadvantage of not allowing the investigation of process-specific working memory functions due to its complexity in regard to the traditional instruments for neuropsychological testing. In this paradigm, sustaining the information online is mixed with processes involved in sequencing, updating, and manipulating the information. Obviously, the simultaneous activation of brain areas comprising a variety of related processes to working memory will confound the differentiation of even well-established correlates such as the human visuospatial working memory (see Fig. 11.6b). Differently, the use of process- or network-specific tasks like the Sternberg paradigm, a delayed match-to-sample task, allows the functional dissociation of working memory components (Gruber and von Cramon 2003). In the first moment a list of stimuli is presented, followed by the presentation of a single stimulus in a second moment. The subject has to respond whether this stimulus has been shown before or not. So, one mechanism frequently used here is the articulatory rehearsal, related to the verbal working memory, eliciting the activation of left-dominant regions, which include Broca, ventrolateral prefrontal cortex (PFC), pre-supplementary motor area (SMA), intraparietal cortex (IPC), and part of the cerebellum (Gruber 2001; Gruber and von Cramon 2003).
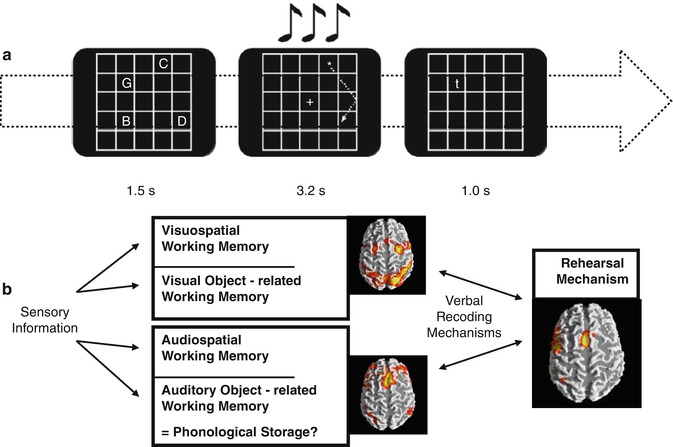
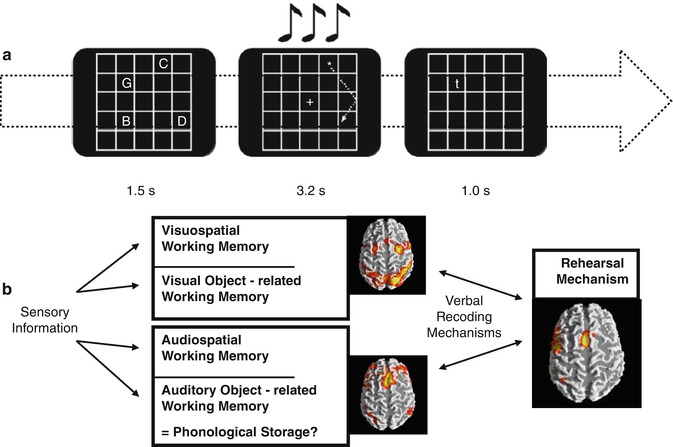
Fig. 11.6
(a) Visuospatial item recognition or verbal item recognition with or without visual or articulatory suppression; visual suppression was performed by following a moving star or articulatory suppression by subvocalizing numbers. (b) Scheme depicting prefrontoparietal and prefrontotemporal circuits according to information domains and the phonological loop (Modified from Gruber and von Cramon (2003), Gruber and Goschke (2004))
The fMRI investigation of non-articulatory mechanisms for sustaining phonological information confirmed the cognitive model (Baddeley and Hitch 1974), describing a dual architecture of the linguistic working memory in terms of a phonological loop. Unlike suggested by lesions that the non-articulatory phonological memory is localized in a single brain area, namely, the inferior parietal lobe, functional imaging studies reinforced the implementation of this mechanism in a bilateral network of several brain regions (Gruber 2001; Gruber and von Cramon 2001, 2003). These mainly include anterior parts of middle frontal gyri and the inferior parietal lobules. Additionally, the functional significance of the anterior middle frontal gyrus for the non-articulatory maintenance of phonological information could be confirmed with the experimental and neuropsychological assessment of patients with lesions in this region (Gruber et al. 2005; Trost and Gruber 2012).
Out of these two partially dissociable and functionally interacting verbal working memory components (Gruber et al. 2007), a visuospatial component could be delineated. fMRI investigation of the latter could consistently show that the frontal eye fields (FEF) and the IPC play a crucial role (Gruber and von Cramon 2003). Working memory neural correlates for object form seem to be differently organized from the visual working memory for spatial information (Gruber and von Cramon 2001, 2003), mirroring the dorsal and ventral pathways of visual processing (see Chap. 9). Overall, grounded in systematic evaluation of the functional anatomy of working memory in humans and converging evidence from research with primates, working memory would be essentially composed of two neurofunctional systems originated from different evolutionary contexts (Goschke and Gruber 2004). From one side is the working memory of different sensory modalities, e.g., visual, auditory, and possibly others, being specifically implemented in this domain, prefrontal-parietal and prefrontal-temporal networks. Considering that these networks are also identifiable in nonhuman primates (Goldman-Rakic 1988, 1996; Romanski et al. 1999), it is plausible that this is an older phylogenetic system (Gruber and Goschke 2004). However, in humans the substantial development of a particular left-dominant language-based system permeated the use of articulatory rehearsal mechanism (Fig. 11.6b) as a predominant working memory tool involved in many higher cognitive functions (Gruber et al. 2000).
11.3.3 Meta-analysis of Executive Functions Including Working Memory
In the previous two sections, many functional studies have been presented and strikingly the neural correlates subserving different executive functions frequently overlapped. Also, lesion studies have supported the existence of a unique correlate behind initiation, inhibition, flexibility, and working memory. However, according to classic cognitive theory, each of these executive functions has been conceptualized as a different and specific domain. As an attempt to present more robust results, the activation likelihood estimation (ALE), a valuable tool to collect and compile imaging information from multiple studies, has been performed (Niendam et al. 2012). Different tasks addressing vigilance, planning, initiation, flexibility, inhibition, and working memory were evaluated as a whole and separately for initiation, flexibility, inhibition, and working memory domains using conjunction analysis in a very large sample (Fig. 11.7a). A common pattern of activation involving the medial and lateral PFC and superior and inferior parietal regions is represented with green and red overlays. Additionally, when results are presented in domain-specific patterns represented by the colors in Fig. 11.7b, the cortical overlapping is even more representative. This reinforces the idea of a general and superordinate cognitive network across different executive domains.
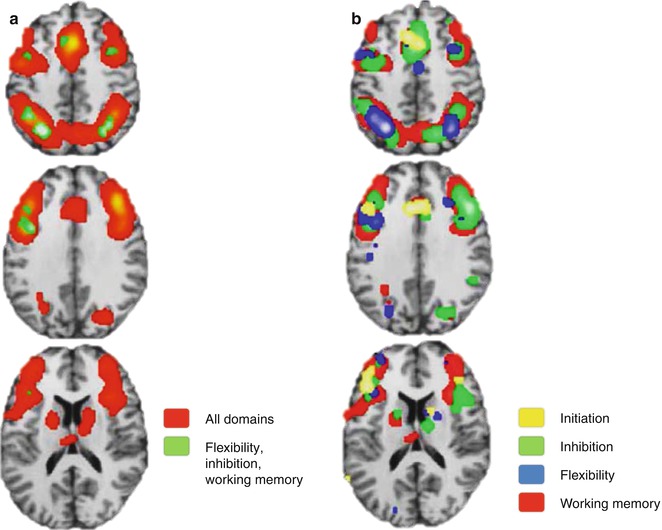
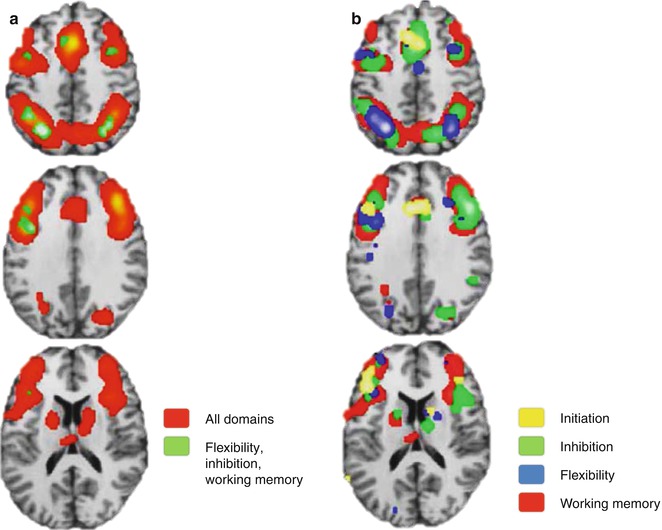
Fig. 11.7
Meta-analytic results supporting a superordinate characteristic of cognitive control network with overlapping results of (a) all studies (red) and the intersection of some domains (green) and (b) separation by domains (Modified from Niendam et al. (2012))
11.3.4 Episodic Memory
Episodic memory is a part of the broad concept of long-term memory. Long-term memory can be generally divided into non-declarative or implicit memory, in which automated motor, cognitive performance, and unconscious associations can be learned, and declarative or explicit memory, in which the content is consciously learned and can be rehearsed and taught. Declarative memory can be further divided into semantic and episodic memory. Semantic memory is the pure factual knowledge about the world, whereas episodic memory is responsible for storing the personal experiences accumulated.
The famous case of the patient H.M. (Scoville and Milner 1957) was a tragic milestone for the comprehension of neural correlates of memory. Due to severe drug-resistant epilepsy, the patient had both hippocampi surgically removed. Since then, immediate rehearsal of information was preserved, but the consolidation into long-term memory was persistently impaired, resulting in permanent anterograde amnesia. This means that it was impossible for H.M. to store new information, only holding it for shorter than a couple of minutes. The hippocampus and extended hippocampal formation are essential for memory consolidation, which was confirmed by behavioral experiments in nonhuman primates. Also, functional imaging studies posed the medial temporal lobe as crucial in storing new content in episodic memory, involved in long-term consolidation and retrieval of information (Brewer et al. 1998; Wagner et al. 1998). Frequently, however, additional activations displayed in subregions of the prefrontal cortex were associated with successful memory consolidation. It has been considered plausible that these regions played an important role in information selection and organization, as well as strategy selection (Fletcher and Henson 2001). Moreover, it should be clearly stated that the neural prefrontal correlates involved in encoding and retrieving episodic memory or general long-term memory are not exclusive. This correlates are also involved in working memory and recognition processes.
In principle, the retrieval of information from long-term memory can be seen as an update of working memory contents and therefore an activation of working memory itself. Consequently it is not surprising for instance to observe the activation of the anterior middle frontal gyrus during information retrieval in the context of both working memory and long-term memory (Gruber 2001; Fletcher and Henson 2001). Although recent imaging literature is full of speculation about the specialization of subregions of the PFC activated during episodic memory tasks with eventual lateralization, it must be emphasized that in the overall view of the empirical evidence, the structures of the medial temporal lobe likely play a more important role in the establishment and maintenance of episodic memory. The hippocampus per se receives information from sensory association areas over the other mediotemporal structures such as the parahippocampal, perirhinal, and entorhinal cortices (Lavenex and Amaral 2000). While the activation of the entorhinal cortex may be sufficient to ascertain familiarity to specific stimuli (Henson et al. 2003), the hippocampus retains an essential role in making associations with existing memory contents, which means processing contextual information (Weis et al. 2004). This feature allows a genuine recognition of someone’s identity beyond the recognition of a familiar face by retrieving additional information such as the name, situation where we met, and what else is known about this person (Fig. 11.8).
A recent ALE meta-analysis (Viard et al. 2012) addressed the variability in the correlates of episodic memory driven by different tasks applied in the laboratory setting. Multiple paradigms exist, generally demanding the subjects to recall past experiences or imagine future events cued by words, sounds, or pictures with personal or generic content (Crovitz and Schiffma 1974). It has been shown that the type of cue, type of task, nature of the information retrieved, and age of the participants contribute for the elicited imaging correlates to vary. This supports the assumption of some pattern of specialization in parts of the hippocampus and medial temporal structures in dealing with stored information. Future studies addressing networking processes behind this specialization, e.g., with high-resolution functional connectivity and/or structural tractography, could extend the comprehension of whether spatial specificities have to do with perceptual specialization or encoding/recall or both.
11.4 The Prefrontal Cortex in Networks
Different methods of analysis of fMRI data allow the investigation of connectivity patterns. Functional and effective connectivity are based, differently from the activity analysis already seen, on the activation patterns along the time or timecourses of certain regions of interest (ROIs). Other regions presenting similar or opposite timecourse patterns, namely, positive or negative correlations, to these ROIs can be identified and are assumed to play a role in the network. Functional connectivity is a very important complementary approach that allows focusing on the investigation of regions that not necessarily present wide and robust activation, but even though might encompass significant functional importance, e.g., parts of the hippocampus. It is valid to reinforce that this field is newer and therefore more speculative, although with persistently increasing interest and importance.
11.4.1 Task-Based and Resting-State Insights
The brain is organized by highly coordinated networks. Positive activations are seen in the dorsal attention and frontoparietal networks (Fig. 11.9) during the performance of external goal-oriented tasks (task-positive), whereas the so-called default network (DN) is generally deactivated (task-negative). Conversely, during rest or introspective cognitive processes such as internal monitoring, the DN is activated, while the other two networks deactivate. The interplay between task-positive and task-negative networks has been shown in many studies (Biswal et al. 1997; Biswal 2012; Fox et al. 2005; Greicius et al. 2003; Margulies et al. 2007; Raichle et al. 2001
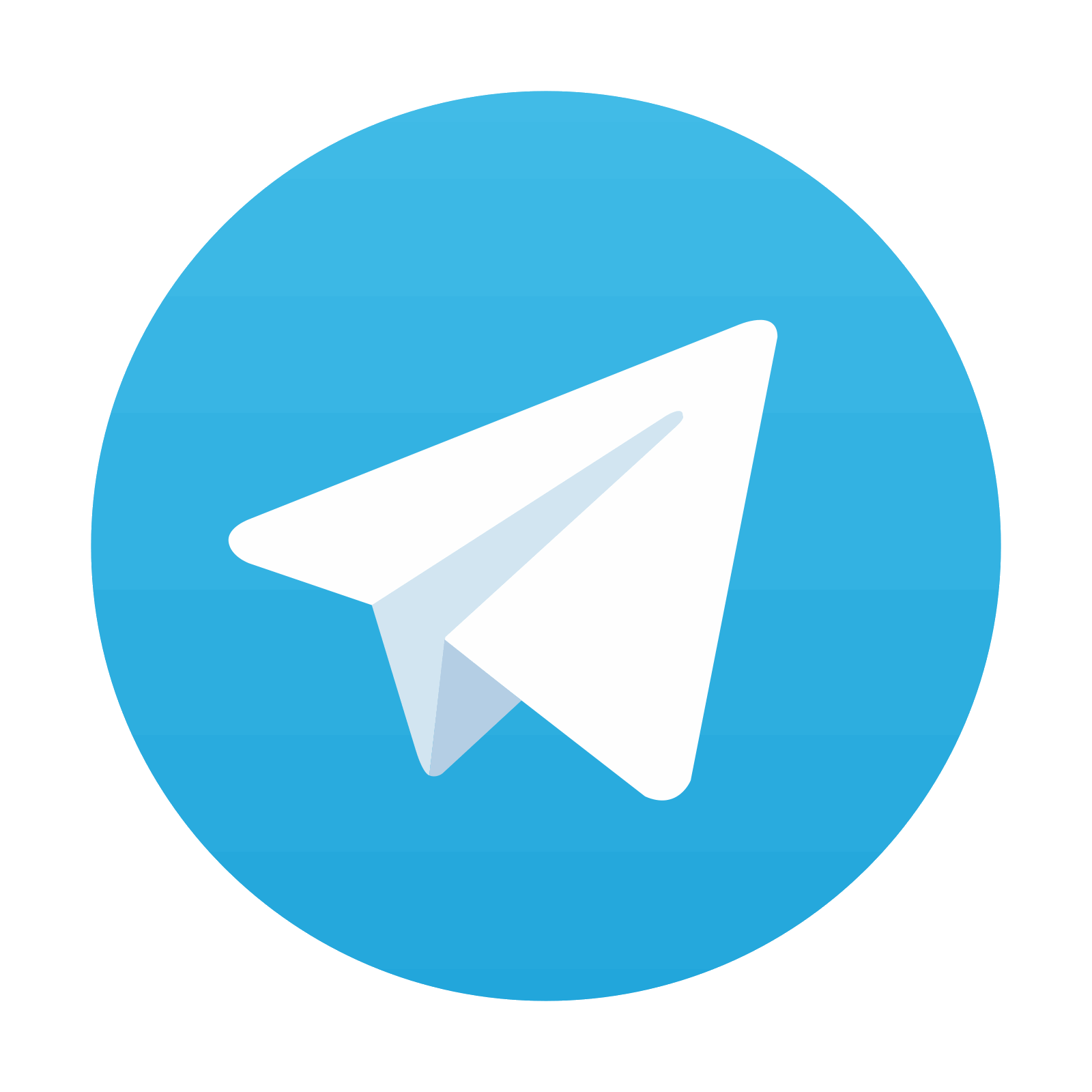
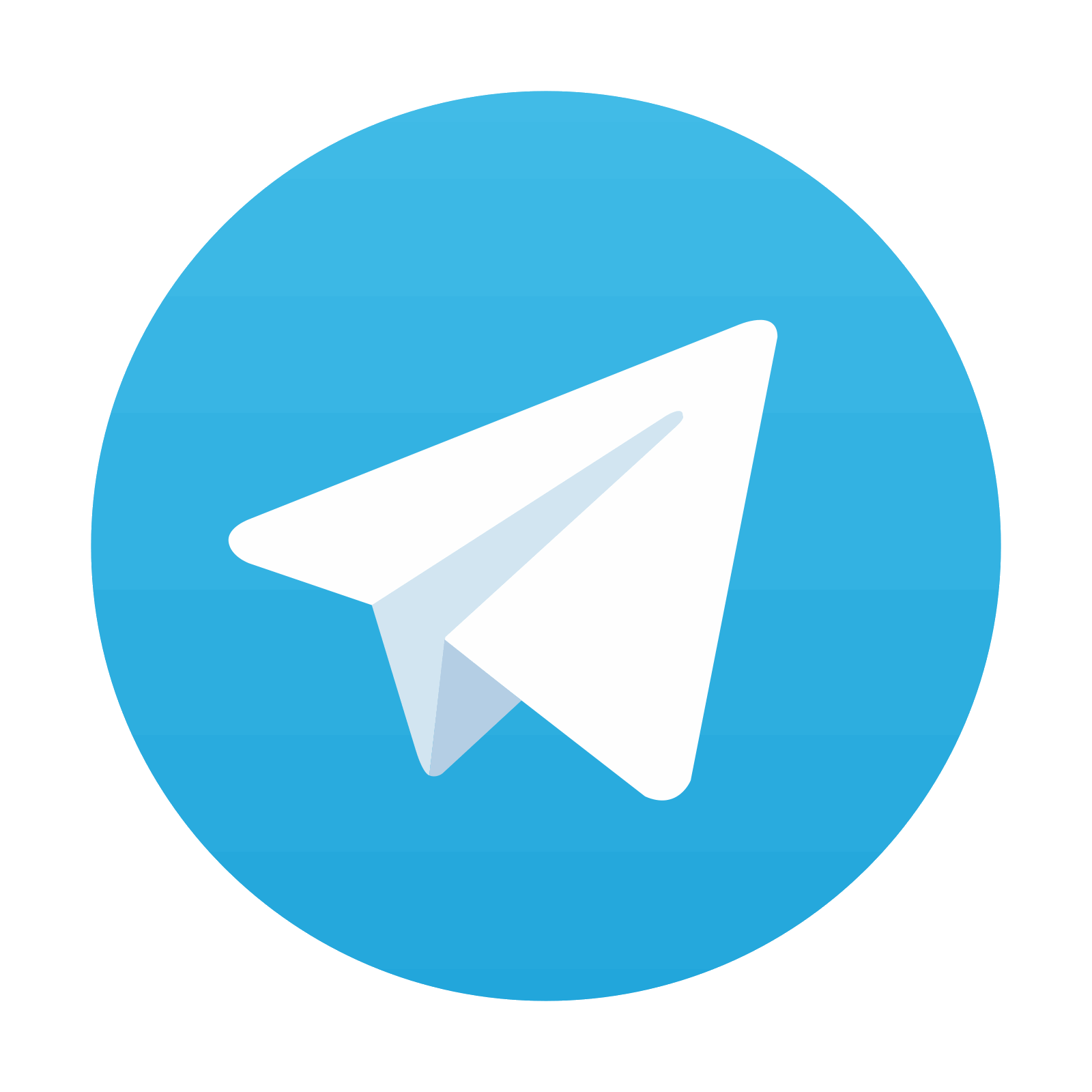
Stay updated, free articles. Join our Telegram channel
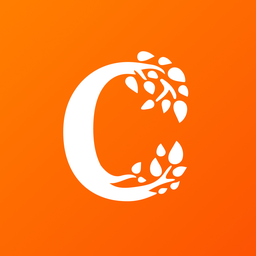
Full access? Get Clinical Tree
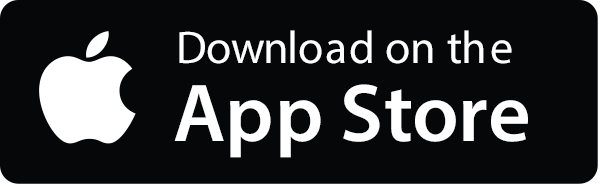
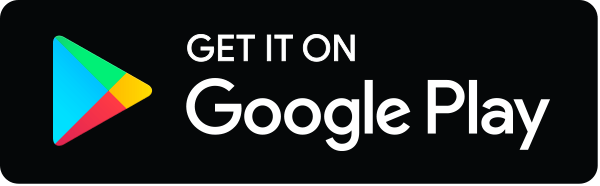