Fig. 1
Atherosclerosisin the carotid bulb from three endarterectomy specimens showing morphological variations. (a) A mature necrotic core with intraplaque hemorrhage (asterisk) is the main component of the plaque. The overlying fibrous cap is an inhomogeneous thickness. (b) The plaque is mainly fibrotic with small areas of lipid accumulation (white arrows) and calcification. (c) Pieces of dense calcification comprise the main portion of the plaque (hollow arrow)
Pathogenesis of Carotid Atherosclerosis
Atherogenesis is now appreciated as a multifactorial process in which the arterial wall interacts with a number of systemic and local factors. The disturbed flow conditions at carotid bifurcation make the carotid bulb a predilection site of extracranial carotid artery for atherosclerosis. Nonetheless, more extensive disease, detectable by improved imaging approaches, is not uncommon, particularly in the common carotid artery. Individual variations in the geometry of carotid bulb and the unknown extent of outward remodeling make it difficult to discern the true plaque burden by angiography or similar techniques.
The accumulation of extracellular lipids and cell debris, the migration and proliferation of smooth muscle cells, and extracellular matrix production are recognized processes in the formation of plaques with well-developed necrotic core(s) and an overlying fibrous cap, or fibroatheroma as described by Virmani et al. in coronary atherosclerosis [6]. Structural changes are paralleled by the recruitment and activation of immune cells that respond to local insult and modulate the inflammatory process. A variety of immune cells including monocytes, lymphocytes, and, more recently, granulocytes have been shown to play a role in the pathogenesis and prognosis of atherosclerosis. Furthermore, it has been suggested through dedicated experimental and human autopsy studies that immune cells can be highly adaptive in response to microenvironmental signals. In accord, functionally indistinct monocytes may differentiate into polarized macrophage subpopulations in the atherogenic milieu, taking on heterogeneous biological functions that may stabilize or destabilize the plaque [7]. Therefore, the biological complexity of plaque inflammation has placed a higher demand on molecular imaging by emphasizing the activities rather than the mere presence of immune cells. Another morphological change that initiates early in the disease process is plaque neovascularization, resulting from the penetration of the medial layer (i.e., tunica media) by hyperplastic vasa vasorum (i.e., the nutritional microvasculature present in large- and medium-sized arteries, with the physiological function of supplying oxygen and nutrients to the outer portion of the medial layer). Initially considered as a compensatory mechanism to alleviate arterial wall hypoxia as wall thickness increases, accumulating evidence from recent studies suggests that plaque neovascularization has an active role in atherothrombosis by contributing to the development, progression, and destabilization of atherosclerotic plaques. Leaky microvessels may facilitate the entry of inflammatory cells as well as plasma proteins. Furthermore, it is thought that episodic ruptures of plaque microvessels are likely the origin of intraplaque hemorrhage, a common finding in advanced human lesions. Improvements in research approaches, for both histopathology and imaging, have recently enabled a better appreciation of the prominent role of intraplaque hemorrhage in atherogenesis as well as the pathophysiology of clinical manifestations. However, mechanistic insights about intraplaque hemorrhage are still limited at this moment, as the phenomenon is rarely seen in prevailing animal models.
Histopathological Characteristics
To date, much of the understanding of carotid atherosclerotic plaque morphology is based on histopathological examination of carotid endarterectomy specimens, which do not cover the full spectrum of lesion severity as compared to human autopsy. The peripheral sections of advanced carotid specimens are presumed to give a glimpse of early or intermediate lesions, which frequently show proteoglycan accumulation, lipid retention, or early stage necrotic cores. Based on these observations, it appears sensible to describe carotid plaque morphology with nomenclature proposed for classifying coronary atherosclerosis. Yet caution should be exercised when making an analogy for a specific feature with regards to its role in pathogenesis and prognosis, given the distinct differences in vessel diameter and flow conditions between coronary circulation and extracranial carotid circulation.
Few studies have attempted to compare plaque morphologies between coronary and carotid atherosclerosis, yet notable differences seem to be present. The fibrous cap overlying necrotic core(s) is at risk of rupture in both coronary and carotid lesions. The high mechanical forces in the carotid circulation may render the fibrous cap of carotid atherosclerosis more vulnerable to rupture, as it has been reported that ruptured carotid plaques have much thicker fibrous cap and fewer macrophage infiltration than ruptured coronary plaques [8]. Thrombotic activity typically ensues, yet the high flow velocity is likely a limiting factor for the propagation of mural thrombus and the healing of fibrous cap rupture. In line with the hypothesis, ulceration is reported more frequently in carotid atherosclerosis than in coronary atherosclerosis, whereas total thrombotic occlusion of carotid stenosis is not as common as in coronary atherosclerosis. Fibrous cap erosion and calcified nodules are the other two types of surface disruption reported in the coronary artery, which may be accountable for over a third of atherothrombotic events in coronary atherosclerosis [6]. However, in the carotid artery, plaque rupture is predominant, while plaque erosion does not seem to be a major cause for atherothrombosis [9]. Although calcified nodules are more frequently seen in carotid atherosclerosis in comparison to coronary atherosclerosis, its role in atherothrombosis in the carotid artery has yet to be determined.
Plaque Morphology and Clinical Presentation
Morphological and pathological features of carotid atherosclerosis have been shown to be associated with downstream neurological ischemic events. Despite the longtime focus of clinical imaging on luminal stenosis, chronic stenosis or occlusion from carotid atherosclerosis rarely leads to ischemic stroke and is therefore not considered the pathological substrate of ischemic stroke. In a study of 269 carotid plaques obtained en bloc from surgical endarterectomy (96 ischemic stroke, 91 transient ischemic attack, 82 asymptomatic), Spagnoli et al. observed a high prevalence of fresh mural thrombus constituted of platelets or fibrin on plaque surface, which was associated with either plaque rupture (90.1 %) or erosion (9.9 %) in patients with stroke [9]. Furthermore, fresh thrombus was more frequently observed when carotid endarterectomy was performed closer to symptom onset. With carotid plaques collected from participants of the North American Symptomatic Carotid Endarterectomy Trial (NASCET) and the Asymptomatic Carotid Atherosclerosis Study (ACAS) trials (71 symptomatic, 170 asymptomatic), Fisher et al. noted that thrombus was associated with plaque ulceration, both of which were more prevalent in symptomatic patients than in asymptomatic patients [10]. Another study by Redgrave et al. examined 570 consecutive symptomatic carotid plaques and demonstrated a continued post-event decline of plaque vulnerability features including cap rupture and plaque inflammation [11]. These studies have provided important insights onto the pathogenesis of ischemic events associated with carotid atherosclerosis. A typical plaque responsible for a recent ischemic stroke can be described as one with active thrombosis on the luminal surface, triggered by plaque rupture and exposure of thrombogenic materials to the flowing blood. Subsequently, acute plaque rupture and thrombosis may lead to artery-to-artery embolism or thrombotic occlusion, which is in line with clinical observations.
Mechanisms of plaque rupture are not yet fully understood. In the study by Redgrave et al. [11], two morphological features demonstrated independent association with cap rupture – intraplaque hemorrhage and inflammatory cell infiltration of fibrous cap. Other studies based on data from coronary atherosclerosis have suggested that fibrous cap thickness and necrotic core size are good discriminators of plaques at risk for rupture from those that are stable [12]. Of note, the degree of stenosis could fit in here as one of the risk factors for plaque rupture, considering the increased mechanical forces associated with local narrowing. Some of these risk factors may have a more dynamic natural course, such as cap inflammation. It is plausible that in vivo characterization of major risk factors of cap rupture may enable accurate estimation of the short-term or long-term risk of neurological ischemic events in patients with carotid atherosclerosis.
MR Imaging Techniques
In contrast to luminal imaging using bright blood MR angiography, vessel wall visualization and the identification of components of atherosclerosis require suppression of signal from intraluminal blood. Thus, MRI sequences commonly referred to as black-blood MRI are essential for vessel wall imaging. Normal extracranial carotids have thin pulsating walls and are often situated in deep tissue planes in patients with a large neck. Carotid bifurcation, the most frequent site of carotid atherosclerosis, has a complex flow pattern with recirculating flow. Thus, complete flow suppression in carotid bifurcation requires special consideration of the flow conditions at carotid bifurcation.
In addition to blood suppression, considerations of spatial resolution, signal-to-noise ratio (SNR), motion suppression, etc., are also important to obtaining diagnostic quality vessel wall images. Atherosclerotic plaque components are small but complicated structures that require high spatial resolution for identification and quantification. Of note, the requirement of high spatial resolution must be achieved with good SNR within a clinically acceptable scan time. Another reason to avoid long scan times is to reduce motion artifacts. Due to the unique location of carotid arteries, patient swallowing has been one of the major causes for degraded image quality of carotid MRI, whereas it will be less of a problem if the scanning time is short.
Contrast-to-noise ratio (CNR) is equally important in order to characterize vessel wall pathology. Delineation of the lumen requires good CNR with adequate blood suppression, while delineation of the outer wall requires good fat suppression. This allows plaque boundaries to be clearly identified and disease burden to be accurately estimated. Furthermore, MRI sequences with special contrast mechanisms are required for identification of various plaque components such as calcification, lipid-rich necrotic core, intraplaque hemorrhage, etc. Indeed, a unique advantage of MR plaque imaging is to employ more than one contrast weightings to help differentiate various atherosclerotic components.
Thus, carotid vessel wall imaging places special technical constraints on field strength, coils, and pulse sequences, which are required for accurate identification of pathology. Carotid MRI protocols need to consider all these aspects while being tailored for each study. This section outlines the established carotid MRI sequences and emerging trends in carotid atherosclerosis imaging.
Field Strength
Initial development of carotid vessel wall MRI took place on clinical 1.5-T scanners. Moving to higher field strengths can increase the available SNR. Initial studies comparing 1.5 and 3-T carotid MRI found an improvement of wall SNR and lumen/wall CNR by 1.5-fold for T1-WI imaging and 1.7–1.8-fold for proton-density-weighted/T2-WI imaging [13]. With optimization of sequences for change in field strength, plaque components can be identified with similar repeatability at 3-T. However, quantification of some components, such as calcification and intraplaque hemorrhage, may differ due to higher susceptibility effects at 3-T. Additionally, the specific absorption rate (SAR) is higher at 3-T and should be accounted for in protocol optimization. Carotid MRI at higher field strengths is challenging due to inhomogeneous excitation, suboptimal fat suppression, and higher susceptibility. Despite these disadvantages, carotid imaging is moving into 7-T due to the advantages of higher SNR.
Coils
At 1.5 and 3-T, body coil transmission is used for homogenous excitation, which is also useful for many blood suppression sequences that use nonselective excitation. However, body coil reception does not provide a high enough SNR for carotid vessel wall MRI within clinically acceptable scan times. Thus, phased array surface coils with a small diameter and applied close to the neck provide improved SNR and are often used for vessel wall MRI. Phased array coils with four, six, and eight coil elements have been designed for carotid imaging. Compared to a four-element design, an eight-element design was shown to provide 1.7-fold higher SNR and larger coverage along the axis of the artery [14]. Some of these coil designs are available commercially on all major scanner vendor platforms. MRI at 7-T also requires custom coil design, but these are currently available for research purposes only.
Multi-slice Black-Blood MRI
Traditionally, the carotid vessel wall is scanned using 2 mm thick slices with higher in-plane resolution of 0.5–0.6 mm. A stack of 12–16 slices centered on the carotid bifurcation covering a 3 cm region is used in a multi-slice black-blood MRI protocol. Blood suppression can be achieved by black-blood preparation of the magnetization prior to gradient or spin-echo acquisition. The sequences used for black-blood imaging can be briefly described as below:
(a)
Spin-echo acquisition: Direct spin-echo MRI without any magnetization preparation can provide black-blood contrast with proper choice of echo time (TE). Only spins experiencing both the excitation and refocusing spins will produce signals. If after experiencing the 90° pulse blood flows through the slice during TE and does not experience the refocusing pulse, it will produce a black-blood effect in the acquired echo. Long TE spin-echo or fast spin-echo sequences such as T2-WI images exhibit this effect. However, slow flow and recirculating flow are not suppressed by this method. Increasing slice thickness will also lead to more flow artifacts.
(b)
Inflow saturation: In this method, the signal from a thick slab proximal to the carotid bifurcation is completely dephased every repetition time (TR). The dephased blood then flows through the imaging slice and produces a black-blood effect. This method can be used for short TE sequences such as T1-WI contrast but is prone to flow artifacts depending on the thickness of the saturation slab and its distance from the imaging slice. A traveling saturation band is used to ensure that the distance to all slices remains the same and a short delay time is used so that blood signal does not recover before being imaged. Advantages of inflow saturation include low SAR, wide availability, and selectivity in suppressing arteries and/or veins.
(c)
Double inversion-recovery (DIR) and variants: DIR preparation consists of two inversion pulses followed by a delay before image acquisition. A nonselective inversion pulse is followed by a slice-selective re-inversion of the imaging slice. Blood with inverted magnetization proximal and distal to the imaging slice flows into the imaging slice while simultaneously undergoing T1 relaxation. The inversion delay time (TI) is calculated such that blood magnetization is zero during image acquisition, thereby causing a black-blood effect. Both arterial and venous blood are therefore nulled by DIR. The long TI time allows sufficient time for blood inflow and ensures good blood suppression. However, artifacts may still occur due to slow or recirculating flow since the non-inverted blood within the imaging slice may not have time for outflow from the imaging slice. Increasing imaging slice or slab thickness tends to worsen flow artifacts with DIR. DIR has better performance than inflow suppression and is widely available on all vendor scanner platforms. Accurate lumen identification in vessel wall imaging requires at least a DIR preparation. The primary disadvantage of DIR for carotid imaging is that it is a single slice method. Multi-slice DIR (MDIR) preparations have been proposed to reduce scan time associated with DIR [15]. The current most efficient method uses a slab-selective re-inversion rather than a slice-selective inversion, thus allowing up to eight slices to be acquired with good flow suppression within a single TR [16].
(d)
Motion sensitization: All the above methods perform best with laminar blood flow with artifacts arising in cases of slow flow, turbulent flow, and recirculating flow. Flow in the normal carotid bifurcation can be recirculating or turbulent, causing plaque-mimicking artifacts on black-blood MRI. Use of motion-sensitized-driven equilibrium preparation (MSDE) can effectively suppress artifacts from recirculating or turbulent flow [17]. MSDE with two refocusing pulses is less affected by B1 inhomogeneity and eddy current effects than MSDE with one refocusing pulse [18]. MSDE is not dependent on inflow of blood into the imaging slice. Hence, it can be used for non-axial slice acquisition. Additionally, MSDE is also compatible with acquisition of thick slabs and is therefore used for three-dimensional (3D) black-blood MRI. An extension of motion sensitization uses a train of delay alternating with nutation for tailored excitation (DANTE) subpulses with interspersed gradient dephasers for suppression of blood flow [19]. DANTE with high amplitude dephasing gradients can also be used for 3D MRI.
3D Black-Blood MRI
MRI studies of atherosclerotic plaque have generally used 2–3 mm thick axial slices, since black-blood techniques such as inflow suppression or DIR rely on inflow or outflow for efficient blood suppression. Compared to the traditional two-dimensional (2D) sequences, 3D black-blood MRI can improve the accuracy and the repeatability of plaque measurements due to higher spatial resolution in the z direction and less partial volume averaging. However, this requires blood suppression in a relatively thick slab, and therefore use of DIR can result in increased flow artifacts. Since MSDE flow suppression does not depend on flow, 3D black-blood MRI can be achieved with high isotropic spatial resolution with this technique. A 3D MSDE prepared rapid gradient echo (3D-MERGE) vessel wall imaging technique can provide 0.7 × 0.7 × 0.7 mm3 isotropic resolution within a 2-min scan time with good blood suppression (Fig. 2) [20]. Since 3D-MERGE datasets are isotropic, interactive reformatting of multiple image planes can be used for plaque visualization. Spin-echo-based sequences with variable flip angle long echo trains have also been suggested for imaging plaque burden [21]. Further studies on the use of 3D sequences for identification of plaque components are required before 3D MRI can be used in a similar way to current 2D MRI protocols.
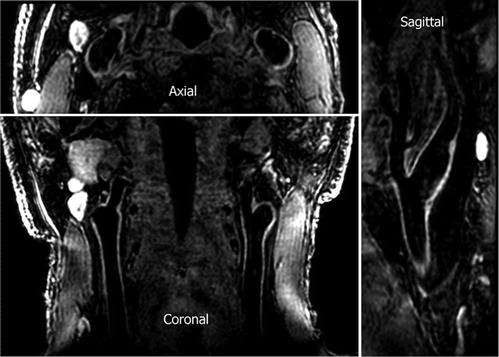
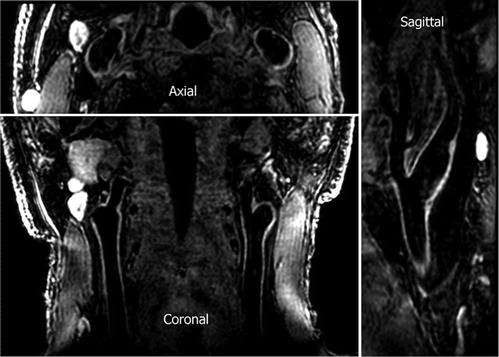
Fig. 2
3D isotropic high-resolution (0.7 × 0.7 × 0.7 mm 3 ) carotid artery images acquired with 3D-MERGE. Two major advantages offered by 3D isotropic black-blood vessel wall imaging are shortened scan times for clinical utility and flexible reformation capability for improved plaque visualization. Note that vessel wall boundaries are clearly visible on all reformats (Reprinted with permission from Balu et al. [20])
Multi-Contrast MRI
Atherosclerotic plaques may range from simple wall thickening to complicated plaques with multiple plaque components. By varying pulse sequence parameters, image contrast may be manipulated such that specific plaque components are visible in each contrast weighting. For example, a highly T1-WI fat-suppressed sequence will show intraplaque hemorrhage with high signal intensity compared to fibrous tissue or muscle. Thus, a multi-contrast MRI protocol where multiple contrast weightings are used in a complementary fashion is essential for comprehensive evaluation of atherosclerotic plaques. Table 1 shows a typical carotid MRI protocol with traditional 2D multi-contrast MRI in comparison to newer isotropic 3D MRI sequences. Single contrast weightings may be used when only a subset of plaque components is of interest. Sequences of interest for detecting important plaque components are briefly described. Table 2 shows the appearance of plaque components on each of the different contrast weightings.
Table 1
Multi-contrast carotid MRI protocol for 3-T
Pre/post-contrast T1-WI | Time-of-flight | T2-WI | Proton-density -weighted | SNAP sequence | 3D-MERGE sequence | |
---|---|---|---|---|---|---|
Sequence | TSE | TFE | TSE | TSE | PSIR | TFE |
Image mode | 2D | 3D | 2D | 2D | 3D | 3D |
Scan plane | Axial | Axial | Axial | Axial | Coronal | Coronal |
TR (ms) | 800 | 20 | 4,800 | 4,800 | 13 | 10 |
TE (ms) | 10 | 4.6 | 50 | 10 | 4 | 5 |
Flip angle (°) | 90 | 50 | 90 | 90 | 11 | 6 |
FOV (cm) | 14 × 14 | 14 × 14 | 14 × 14 | 14 × 14 | 14 × 14 × 3.2 | |
Resolution (mm) | 0.55 × 0.55 | 0.55 × 0.55 | 0.55 × 0.55 | 0.55 × 0.55 | 0.7 × 0.7 × 0.7 | 0.7 × 0.7 × 0.7 |
Slice thickness (mm) | 2 | 1 (interpolated) | 2 | 2 | Not applicable | Not applicable |
Blood suppression | QIR/MSDE | Venous inflow suppression | DIR/MSDE | DIR/MSDE | Not applicable | MSDE |
Fat suppression | Yes | No | Yes | Yes | Yes | Yes |
Table 2
Plaque component classification using multi-contrast MRI
Time-of-flight | T1-WI | Proton-density-weighted | T2-WI | Post-contrast T1-WI | |
---|---|---|---|---|---|
Lipid-rich necrotic core | o | o/+ | o/+ | −/o | − |
Intraplaque hemorrhage | + | + | −/o | −/o | −/+ |
Calcification | − | − | − | − | − |
Loose extracellular matrix | o | −/o | + | + | + |
Dense fibrous tissue | − | o | o | o | + |
Lipid-Rich Necrotic Core
Lipid accumulation in plaques evolves from lipid pools to necrotic cores containing cell debris with progression of atherosclerosis. Fat suppression used for plaque imaging suppresses signal from triglycerides in subcutaneous fat but does not suppress signal from necrotic core, where the signal mainly originates from free cholesterols and cholesteryl esters. Necrotic core is isointense on T1-WI sequences, but can be seen on T2-WI sequences as a hypointense region due to its short T2 components. Thus, a non-contrast MRI protocol requires a T2-WI sequence for detection of necrotic core.
Sensitivity and specificity for detection of necrotic core can be improved by administration of gadolinium contrast agent such as gadopentetic acid (Gd-DTPA) [2]. Due to necrotic contents, gadolinium uptake into necrotic core is negligible compared to surrounding fibrous tissue. Post-contrast difference between necrotic core and fibrous tissue is maximal 5–10 min after contrast injection. Therefore, necrotic core can be readily identified by comparing pre-contrast and post-contrast black-blood T1-WI images. However, adequate blood-suppression post-contrast is challenging. The T1 of blood decreases sharply after contrast administration, but the change in blood relaxivity is not predictable since it is dependent on distribution volume – an unknown patient-dependent factor. Thus, while black-blood techniques such as DIR provide good blood-suppression pre-contrast, adjustment of TI of DIR post-contrast is empirical and does not provide adequate blood suppression in all subjects. A quadruple inversion-recovery (QIR) preparation has been introduced for this purpose [22]. QIR consists of two DIR modules in tandem and consists of two TIs that are adjusted such that blood suppression is achieved for the set repetition time (TR) of the sequence. QIR blood suppression is T1 insensitive, thus enabling the same sequence parameters to be used for both pre- and post-contrast T1-WI MRI.
Intraplaque Hemorrhage
Bleeding into the plaque may come from either the lumen secondary to surface rupture or from the vasa vasorum originating from the adventitia. Hemoglobin in the red blood cells then passes through several stages of degradation. One of the intermediate stages is the formation of highly paramagnetic methemoglobin. Methemoglobin is visible as hyperintense regions on T1-WI images such as T1-WI fast spin echo or time-of-flight images. However, the sensitivity and specificity for detection of intraplaque hemorrhage can be improved by use of highly T1-WI sequences [23].
Magnetization-prepared rapid gradient echo (MPRAGE) is often used for detection of intraplaque hemorrhage [3]. The MPRAGE sequence employs an inversion recovery preceding a gradient echo acquisition. Fat suppression is also employed to reduce signals from subcutaneous fat. MPRAGE has been shown to have higher sensitivity and specificity for intraplaque hemorrhage detection than T1-WI fast spin echo or time-of-flight. However, blood suppression in MPRAGE can be challenging since TI has to be optimized simultaneously for both blood suppression and visualization of intraplaque hemorrhage. A better approach combines MPRAGE acquisition with phase-sensitive reconstruction (PSR). While PSR requires the additional acquisition of a reference image, it provides additional benefits over blood suppression. PSR is used in the simultaneous non-contrast angiography and intraplaque hemorrhage (SNAP) MRI sequence where the nonselective inversion pulse of MPRAGE is replaced with a slab-selective inversion [24]. The TI of SNAP is adjusted such that blood flowing into the imaging plane is inverted only a single time compared to the blood in MPRAGE that may be inverted multiple times. During image acquisition at TI, flowing blood which has a long T1 has negative longitudinal magnetization, while short T1 components such as intraplaque hemorrhage have a positive longitudinal magnetization. PSR of SNAP takes advantage of this difference in sign of longitudinal magnetization to provide two images from the same acquisition: negative part provides an angiogram, while the positive part provides signal for intraplaque hemorrhage. Figure 3 shows an ulcer on the SNAP angiogram with corresponding intraplaque hemorrhage in the plaque. Since both MR angiography and intraplaque hemorrhage imaging are obtained from the same SNAP acquisition, they are naturally registered together.
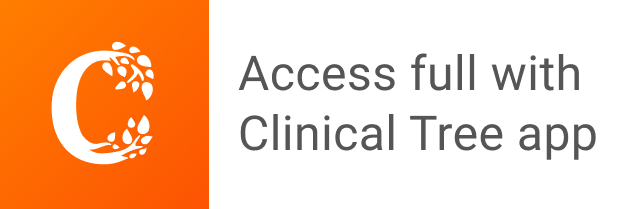