Introduction
Magnetic resonance angiography (MRA) enables noninvasive assessment of vascular disease in both the arterial and venous circulation. Owing to the availability of volumetric data sets without the need for ionizing radiation exposure and facilitated by technologic and methodological advances in hardware, data sampling, and image reconstruction approaches, MRA has become a powerful tool for the diagnosis of a variety of vascular pathologies. MRA techniques explore different mechanisms for contrast generation and can provide clinical information on vessel luminography or function. The method of choice depends on the diagnostic task and the specific demands of the vascular territory.
Time-of-flight (TOF) and phase-contrast (PC) MRA differentiate the signal in vessels from surrounding tissues based on inflow effects (TOF) or velocity encoding (PC) of the moving blood. The latter can be used to actually quantify velocities and blood flow. Balanced steady-state free precession (bSSFP) has been recently added to the repertoire of clinically used MRA techniques and generates vascular maps based on a T2/T1-weighted image contrast that can be enriched by using concepts of inflow encoding and signal saturation. TOF, PC, and bSSFP MRA are referred to as non–contrast enhanced (NCE) MRA techniques, since they do not require any external contrast agents.
Yet another very commonly used approach is contrast-enhanced MRA (CE MRA), which uses an intravenous injection of a T1-shortening, gadolinium (Gd)-based contrast bolus. The much higher signal in the blood pool combined with relatively short acquisition times results in a very robust acquisition that provides large anatomic coverage, low sensitivity to artifacts, and short scan times.
This chapter will present the basic physical principles and imaging strategies related to the most common approaches for MRA. The benefits and disadvantages of the various approaches will be illustrated, and considerations for physiologic gating to the cardiac and respiratory cycle will be discussed.
Basic Gradient Echo Sequence
Ideally, all MR data are acquired while in a constant relaxation state, with high spatial resolution, a high signal-to-noise ratio (SNR), ultrashort imaging time, and no artifacts. The difficulty in achieving all these properties has led to the development of many acquisition methods that differ in image contrast, acquisition speed, SNR, susceptibility to and type of artifacts, energy deposited in the imaged patient, suppression of unwanted signal such as fat, and other aspects. Their corresponding images represent a combination of tissue-specific parameters T1, T2, and proton density ρ and scan-specific parameters such as repetition time (TR), echo time (TE), flip angle, field of view (FOV), spatial resolution, magnetization preparation, and so forth.
All MRA imaging strategies described here rely on gradient echo acquisitions for image generation, which have been introduced in Chapter 3 . Gradient echoes allow for faster scanning compared to spin echo acquisitions, owing to the potential for short TEs and short TRs, formed using fast switching of gradients to facilitate a measurable echo formation. The pulse sequence diagram (PSD) for a basic gradient recalled echo (GRE) sequence without phase encoding is shown in Figure 4-1 . After radiofrequency (RF) excitation of the slice (two-dimensional [2D] acquisition) or volume (three-dimensional [3D] acquisition) of interest with a flip angle α less than 90 degrees, a negative readout gradient lobe dephases the transverse magnetization and then refocuses it with a second lobe of inverted polarity, also referred to as the readout gradient, to form a gradient echo at the echo time TE. Data are acquired during the data acquisition window while the readout gradient is played out. Unlike spin echo sequences, this echo is not characteristic of T2 relaxation but rather of T2* relaxation, because dephasing from chemical shift, magnetic susceptibility, and B0 inhomogeneity cannot be inverted by the gradient reversal.
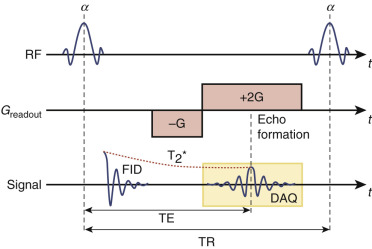
With a long repetition time (TR ≫ T2), there is no carryover of transverse magnetization between excitations because the transverse magnetization is completely dephased. In fast GRE imaging, the TR is significantly reduced and generally less than the T2 values of biological tissues. Under this condition, the transverse magnetization of a preceding RF pulse is not completely dephased and generally results in a complex superposition of free induction decays (FIDs), primary echoes, stimulated echoes, and secondary and higher-order echoes for a train of periodic RF pulses. Under certain conditions, a steady state can be reached from repetition to repetition for one or more components of the magnetization. It is necessary to enforce a steady-state condition for the transverse magnetization in order to acquire data with consistent signal from one excitation to the next. As we will see below, the spoiled gradient echo sequence and the bSSFP sequence are such implementations and form the basis of all MRA sequences discussed here.
Contrast-Enhanced MRA
In CE MRA, contrast between blood and background signal is achieved through the injection of a T1-shortening contrast agent. Although intraarterial injections similar to conventional x-ray angiography are possible, practically all CE MRA exams are conducted with intravenous injections, which dramatically decreases risks and costs as well as improves patient comfort. The agent is extracellular and is typically based on gadolinium, a paramagnetic metal that decreases both T1 and T2 relaxation time. Pure Gd is highly toxic, so clinically used formulations must be chelated to ligands for safe delivery to human patients. Recently the use of Gd-based contrast agents in patients with severely compromised kidney function has been linked to the occurrence of nephrogenic systemic fibrosis (NSF). Proper screening of patients at risk through measures of glomerular filtration rate and serum creatinine level minimizes this risk and provides a high safety profile.
Imaging Basics
Image contrast in CE MRA is explored by the use of heavily T1-weighted sequences. The mixing of the contrast agent with the blood pool will generate high (bright) signal in the vasculature while most other tissues have a much longer T1 relaxation time and appear dark.
The spoiled gradient echo sequence is well suited for this imaging task when played with a short TR. It is designed so that the transverse magnetization generated by an excitation pulse only contributes to the signal acquired in the echo period immediately following this pulse. Therefore these sequences are also referred to as longitudinal steady-state sequences, because only the longitudinal component of the magnetization is in a steady state. In case of ideal spoiling, the transverse magnetization M xy would be completely destroyed immediately after each repetition. In practice this cannot be achieved, but it is possible to almost eliminate the signal contributions from all preceding pulses to the transverse magnetization M xy for each TR. The phase ϕ of both the excitation pulse and the receiver is altered in a pseudorandom sequence to eliminate contributions caused by transversal magnetizations from previous excitations. In addition, a dephasing gradient is played out, usually along the slice-encoding direction after the acquisition of each echo to dephase the transverse magnetization. The dephasing gradients need to be strong enough to ensure a minimal dephasing of 2π of the spin ensembles in all voxels for a homogeneous signal across the images. Figure 4-2 shows the timing diagram for the 3D spoiled gradient-recalled echo (SPGR) pulse sequence.
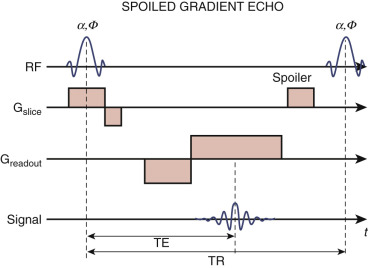
In spoiled gradient echo imaging for CE MRA, the TR values are reduced to less than 10 milliseconds. If the echo time is also very short (TE ≪ T2*), it can be shown that the signal only depends on TR, T1, and α. For large flip angles and a short TR, the sequence then becomes heavily T1 weighted as desired for this application. Vendor implementations and acronyms of this sequence include (Spoiled GRASS = Spoiled Gradient Refocused Acquisition in the Steady State, GE Healthcare, Waukesha, WI), FLASH (Fast Low Angle Shot, Siemens Medical Systems, Erlangen, Germany) and FFE (Fast Field Echo, Philips, Best, The Netherlands). Some publications have been dedicated to assist the interested reader in listing and comparing acronyms for MR pulse sequences.
One tissue that can interfere with the CE vascular signal is fat, which also has a relatively short T1 time. In many parts of the body, high amounts of fat can be present in background tissue and may diminish vascular visualization from CE MRA, especially in the commonly used projection images that represent the whole image volume from various angles. Fat suppression pre-pulses or subtraction of precontrast image masks can be used to enhance the delineation of arteries in such cases.
Bolus Timing.
Intravenous delivery of the contrast can be performed manually, but the method of choice is use of a power injector with a controllable injection rate (in mL/sec) followed by a saline flush. Dosing is typically based on body weight (e.g., 0.2 mmol/kg) but may also be a fixed volume. The injection rate, dosing, and acquisition timing must all be tailored to the vascular territory of interest and to the suspected pathologic condition.
An extremely important aspect of CE MRA lies in the timing of MR acquisition with what is known as the first-pass of contrast through the arteries of interest after the bolus has passed from the venous injection site to the heart and through the pulmonary circulation ( Fig. 4-3 ). There will be a brief time period where the arterial signal is at its peak without any signal in the veins. After this arterial first-pass, contrast will circulate through the venous side, eventually dispersing into background tissue for extracellular Gd chelates. Timing of the acquisition to the first-pass window therefore will best delineate arterial signal from background signal and venous interference.
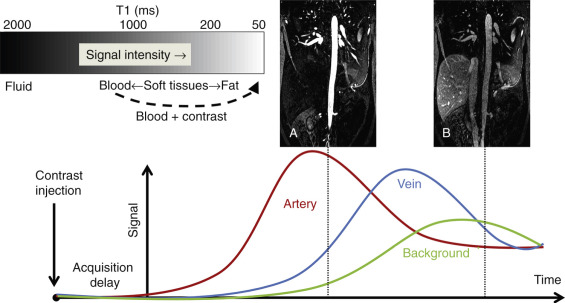
Two main methods of determining this window of first-pass capture are typically used clinically. The first employs a test bolus of a small amount of contrast (2-3 mL) and a subsequent saline flush coupled with a dynamic series of a low-resolution single slice images in the main artery of interest. Saturation slabs above and below the slice of interest can be applied to remove inflow effects. This allows for manual measurement of the contrast arrival times in arteries, veins, and background tissue, so that the main acquisition can be timed accordingly.
The second method uses a technique similar to fluoroscopy for either a manual or automated trigger to start the CE MRA acquisition. For example, manual visual inspection of contrast traversing vasculature in a single thick slice is monitored using continually updated images, usually with a temporal resolution of around 1 second. The acquisition is triggered by the operator, who watches the arrival of contrast. For cases with unusually fast or slow flow, this technique provides the advantage of giving the operator direct feedback on flow characteristics. The thick slice also provides dynamic information for large anatomic coverage. In the automatic triggering method, which is used less frequently, a single voxel or region of interest within the artery is monitored and the CE MRA scan is triggered when this value rises above some predetermined threshold value. The advantage with this method is the removal of user dependence; however, acquisitions for extremely slow flow will not be correctly delayed with this technique.
CE MRA acquisitions in the abdomen are complicated by the additional need for a breath hold that is synchronized to the bolus arrival and data acquisition. The approaches are similar to the ones just described, but the time lost for the initiation of a breath hold can lead to nonoptimal acquisition windows past the peak enhancement or with enhancement in venous structures.
Scan Time Considerations.
Many technical aspects of CE MRA must be correctly prescribed and monitored for a successful CE MRA scan completion. Imaging parameters are chosen to optimize the conflicting aims of high spatial resolution, short scan times, and bright signal of arterial arrival from contrast injection ( Fig. 4-4 ). Because the primary target of a CE MRA exam is to capture enhancement during the arterial window, acquisition of the 3D volume should be performed as quickly as possible. In vascular regions of short turnaround between bolus arrival in the arteries and veins (e.g., kidneys, brain) or in pathologic conditions such as arteriovenous malformations (AVMs), this window may be as short as 5 seconds. Consider a 3D renal CE MRA acquisition with an in-plane matrix of 256 × 256 and 64 slices with a typical TR of 5 milliseconds. Without the use of any accelerated imaging, the scan time for such an acquisition would be T = TR × N phase × N slices = 5 milliseconds × 256 × 64 = 82 seconds.
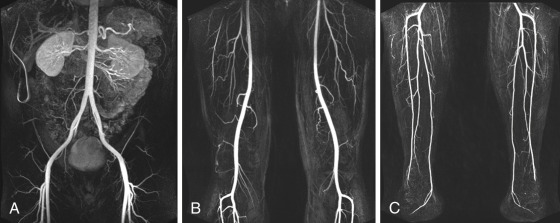
This obvious discrepancy between desirable (and clinically necessary) spatial resolutions and the corresponding scan times has led to incorporation of many innovative rapid imaging approaches to CE MRA, including partial Fourier acquisitions, parallel imaging, and others, many of which have been discussed in Chapter 3 . Parallel imaging uses overlapping sensitivity regions from neighboring coils to undersample data, thus providing massive scan-time savings that come at the cost of reduced SNR. However, CE MRA usually provides ample SNR so that these scan-time savings can be exploited and have proven to be very beneficial.
In addition, k-space properties have been explored to further tailor acquisitions to the properties of CE MRA exams. This includes the use of elliptical centric view ordering, which is designed to capture the center of k-space during peak enhancement when the main contrast of the imaging scene is defined. The higher spatial frequencies that define the peripheral portions of k-space are acquired at time points where decreased arterial signal and potential venous enhancement has less of a detrimental effect on the MRA. State-of-the-art CE MRA sequences combine parallel imaging and high receiver counts with some of the other methods mentioned earlier to reduce scan time to within a breath hold or even shorter durations for dynamic imaging. Potential pitfalls of these CE MRA approaches include improper timing when the acquisition is started too early (no contrast bolus yet) or too late (suboptimal arterial signal and venous overlap).
Time-Resolved CE MRA
The goal of time-resolved CE MRA is to capture multiple time frames of the image volume before, during, and after the passage of the bolus, similar to dynamic series in conventional x-ray angiography, with the added benefit of a 3D acquisition. A great benefit of such an approach is to accurately capture the peak arterial window in cases where synchronization of bolus arrival and acquisition timing are difficult. Other benefits include the ability to visualize complex flow patterns that do not have a single optimal time frame, such as asynchronous enhancements in regions with poststenotic and collateral vascular filling, as well as signal enhancement in complex vascular pathologies such as large aneurysms, dissections, or AVMs. These acquisitions are necessarily performed in even shorter times than single-acquisition CE MRA, and thus spatial resolution must be further compromised or additional temporal constraints must be used in the reconstruction process to facilitate sufficiently high temporal resolution.
Many innovative imaging approaches for rapid MRI have been motivated by and were often first implemented in CE MRA, including keyhole imaging, time-resolved imaging of contrast kinetics (TRICKS), kt-acceleration, and compressed sensing. In keyhole imaging, the acquisition is separated into two phases. In the first phase, only a small central region of k-space is acquired repeatedly and rapidly to capture the dynamic changes during contrast passage. In a later phase, all high spatial frequencies are acquired when the contrast is in the arteries and veins. Complete data sets are reconstructed for all dynamic phases by sharing the high spatial frequencies among all time frames. The assumption at work is that most of the signal evolution will be reflected only in the low spatial frequency components near the center of k-space. This approach can generate high temporal resolution but can hide dynamics or late enhancements in certain conditions. The 3D TRICKS approach and similar variants combines keyhole imaging with view sharing and variable rate sampling and partial Fourier imaging to overcome some of these pitfalls and are commonly used in clinical practice. Figure 4-5 shows an example for a dynamic CE MRA of a patient with an aortic dissection, illustrating the benefits of a data series when no single time frame is optimal to properly capture all details. Chapter 5 will further discuss dynamic imaging with CE MRA in the context of capturing tracer kinetics.
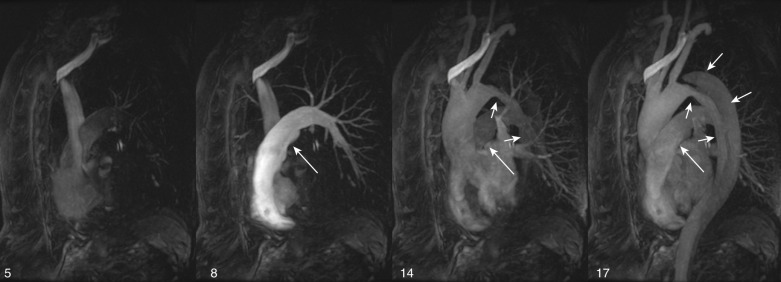
NCE MRA
NCE MRA techniques have been realized since the advent of the clinical use of MRI. There are several advantages and disadvantages when comparing NCE MRA with CE MRA. CE MRA usually provides higher signal and contrast and is considered more robust in many vascular territories. However, NCE MRA does not require injection of a contrast agent, thereby reducing costs and increasing patient safety, especially for patients with renal insufficiency at risk for NSF. In addition, scan times can be extended beyond an arterial window, thereby facilitating higher resolution scans or physiologic gating to the respiratory and/or cardiac cycle, which are usually time-prohibitive in CE MRA. As will be shown, PC MRI can also provide quantitative flow measurements for added value to the diagnostic process.
Recent advances in MR hardware, such as multireceiver coil arrays and parallel imaging, as well as gradient performances that facilitate the use of bSSFP imaging in addition to the search for alternative MRA approaches for patients at risk for NSF, have renewed interest in NCE MRA techniques over the last 15 years. This, coupled with the development of more advanced gradient hardware and the introduction of higher-strength magnetic fields in clinical practice, has greatly increased the use of NCE MRA techniques clinically. This section will highlight the imaging principles behind the most common NCE MRA approaches: TOF, PC, and bSSFP MRA.
Time-of-Flight MRA
TOF MRA is a commonly used technique, especially in the peripheral and intracranial vasculature. As in CE MRA, the pulse sequence employed is a spoiled gradient echo sequence, here with slightly longer TRs (<50 ms) as shown in Figure 4-6A . The repeated slice-selective RF pulses in a spoiled gradient echo sequence diminish signal from stationary tissue until equilibrium is reached from one excitation to the next. However, blood flowing into the imaging slice experiences no or very few of these RF pulses, which results in high blood signal relative to the saturated static tissue. Thus the contrast in TOF is dependent on the inflow of unsaturated (often called “fresh”) blood and the proper suppression of background tissues within the imaging slice. Consequently the slice or slab thickness as well as the speed of the blood flow play a crucial role for image contrast (see Fig. 4-6B ). The slower the blood flow and the wider the imaging slab, the more the contrast diminishes. Perpendicular orientation of the imaging slice to the flow direction generates the highest image contrast, whereas in-plane blood flow diminishes vessel contrast. As a result, TOF angiography is best suited for territories with mostly straight vessel paths.
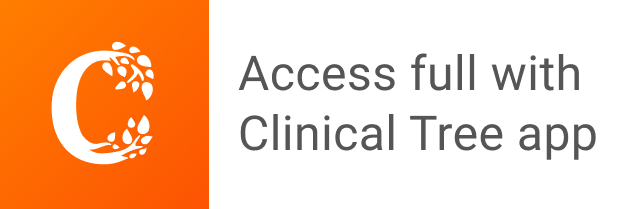