Instrumentation: Magnets, Coils, and Hardware
Luca Marinelli
John F. Schenck
Douglas A.C. Kelley
Introduction
In Würtzburg, Bavaria, on November 8, 1895, Wilhelm Röntgen detected a new form of radiation coming from a cathode ray tube he was studying (1). By early January 1896, this discovery of x-rays had been reported in American newspapers and elsewhere, and by the end of that month equipment already available in the MIT Physics Department had been used to confirm Röntgen’s discovery. Clinically useful x-ray images were produced almost immediately, and F.H. Williams presented a live demonstration of skeletal imaging to a Boston medical society in April 1896 only 6 months after Röntgen’s discovery (2).
Compare this with the 40 years that elapsed between the initial proposal of nuclear magnetic resonance (NMR) and the production of the first clinically relevant magnetic resonance (MR) images. The contrast between the time and effort required to develop x-ray imaging and that required to develop MR imaging (MRI) is an indication of the inherent complexity of MR basic science and technology. The Dutch physicist C.J. Gorter first proposed the NMR concept in 1936 (3). However, it was not experimentally demonstrated outside of vacuum chambers until the work of Bloch, Purcell, and their associates shortly after World War II (4,5,6,7,8). The initial applications of NMR concerned basic science research on the physical and chemical properties of matter. The early experimenters used either test tube–sized samples or beams of atomic or molecular particles traveling through evacuated chambers. For the most part, the magnets used were relatively small devices, available as standard equipment in the physics laboratories of the time. These basic science studies engaged some of the most prominent physicists and chemists of the postwar period, and it is interesting that many of these leaders initially doubted the practicality of human imaging (9).
Between World War II and the 1970s, a few NMR studies on human or animal tissues were reported (10,11,12,13,14). However, the key to the modern clinical use of NMR was Paul Lauterbur’s 1973 suggestion (15) that operator-controlled magnetic field gradients could be used to encode position-dependent information in the NMR signal. This suggested the possibility of generating cross-sectional anatomic images of human beings. Several years elapsed before two groups, working more or less concurrently, at Nottingham University produced the first images of human anatomy in 1976 and 1977 (16,17,18). The development of a new category of magnets capable of creating very strong, very uniform, and very stable magnetic fields over an unusually large volume was an early requirement. In other words, it was necessary to scale the NMR apparatus up from a size designed to deal with samples in test tubes to a size capable of accommodating the human body (19,20). When such magnets became available in the late 1970s and early 1980s, experimenters were quickly able to generate useful NMR signals from an entire human head or torso.
The NMR signal produced by a complex source, such as the human anatomy, is an intricate time-dependent voltage trace, which cannot be deciphered by human inspection. The information content of these signals is determined by an elaborate set of time-dependent currents supplied to an array of specialized transmitter and receiver coils that are located, along with the patient, inside the magnet. Several methods for controlling these coil currents and converting the resulting NMR signals into images were proposed during the early development of the field. These suggested methods included the back projection (15), sensitive point (21), and field focusing (22) techniques. However, the vast majority of clinical scanning has been carried out using the Fourier techniques that were originally developed for NMR spectroscopy (23) and that were later adapted to imaging (24,25). In its most commonly used form, this technique has become known under the name of spin-warp or spin-echo imaging (26).
Despite the fact that MRI can now be considered a well-developed and well-accepted medical technology, intense technical development efforts and extensive clinical application studies continue to be conducted with the goals of reducing the costs of MRI and increasing its technical capabilities and the number of clinical indications for its use. This has resulted in the availability of a wide range of system configurations and price levels to meet the needs of differing clinical situations.
To protect human subjects during the development and use of medical devices, the US Congress in 1976 amended the Food, Drug, and Cosmetic Act of 1938 to cover the introduction of new medical devices. In response, the U.S. Food and Drug Administration (FDA) issued regulations in 1980 that applied to manufacturers of new medical devices and to researchers using these devices. The regulations are analogous to those that control the introduction of new drugs in the United States. They require the generation of data on the safety and efficacy of new medical devices before they can be marketed. MRI was the first medical imaging modality subjected to these regulatory requirements (27,28,29).
This chapter considers the main magnet and the gradient and radiofrequency (RF) coils that are unique to MRI scanners. It also discusses the computer hardware required by these devices. The chapter emphasizes the application of MRI to proton imaging, which has been by far the most important clinical use. Other applications, such as the spectroscopy of phosphorus, carbon, or other nuclei, involve generally similar instrumental considerations. A single chapter can provide only
an overview of the technical aspects of MR scanners. Several book-length accounts are available (30,31,32,33,34,35,36,37,38).
an overview of the technical aspects of MR scanners. Several book-length accounts are available (30,31,32,33,34,35,36,37,38).
Impact of MRI in Medicine and Technology
The development and widespread clinical utilization of MR scanners during the 1980s represented a technical tour de force. Engineering advances were combined with newly developed scientific and clinical understanding and with large financial investments to produce a completely new modality for the field of diagnostic imaging. It was possible to control the data acquisition process and to compute the resulting images in a reasonable time because of tremendous advances in computer technology. To avoid confusion with the field of nuclear medicine and also to avoid the negative connotations often associated with the word nuclear, the phrases nuclear magnetic resonance and NMR, which are commonplace in physics and chemistry, were not taken over into clinical applications. Instead, the presumably more agreeable phrases magnetic resonance imaging and MRI became universally accepted names for this clinical modality. Despite the inherent complexity of NMR, the technology was rapidly disseminated into the practice of medicine once human-size systems became generally available. Although precise numbers are not available, marketing studies suggest that, as of 2014, more than 65,000,000 clinical examinations are being performed worldwide each year. This indicates that more than 850,000,000 diagnostic MR studies were performed between the introduction of clinical MRI in the early 1980s and the end of 2014.
The impact of MRI on clinical medicine is often cited as an example of the benefits to the society of basic research in physics and chemistry. Table 1.1 lists important scientific and technical advances that were crucial to the eventual development of MRI scanners. Also listed are the names of some of the investigators and approximate dates for these developments. It is, of course, not possible to acknowledge in a single table all of the contributors to the development of MRI. The purpose of the table is to provide orientation concerning the large number of basic science advances that were required before MRI could be developed and to emphasize how recently much of this basic understanding has been achieved. It is interesting to note that in the 1980s many elderly patients who were scanned by MRI had been born before the magnetic properties, or even the existence, of atomic nuclei were known.
TABLE 1.1 Some Technical and Scientific Milestones in MRI | ||||||||||||||||||||||||||||||||||||||||||||||||||
---|---|---|---|---|---|---|---|---|---|---|---|---|---|---|---|---|---|---|---|---|---|---|---|---|---|---|---|---|---|---|---|---|---|---|---|---|---|---|---|---|---|---|---|---|---|---|---|---|---|---|
|
In 2001, a group of 225 physicians were surveyed and selected MRI, along with computed tomography, as the most important medical innovation in terms of advancing patient care in the previous 25 years (39). These results were particularly significant because (i) the surveyed physicians were not radiologists, who would logically be expected to be impressed by techniques that had changed their practices in major ways, but, rather, general internists, who ranked the innovations based on their impact on patient management, and (ii) the 25 years over which the innovations were evaluated had seen the introduction of many revolutionary medical technologies such as coronary artery bypass surgery, new treatments for depression, and so on.
The early history of MRI has generated a degree of controversy regarding the priority of various discoveries and inventions and the ultimate capabilities of the technique (40,41). In 2003, many years after their original publications, the Nobel Prize in Physiology or Medicine was jointly awarded to Paul Lauterbur and Sir Peter Mansfield “for their discoveries concerning magnetic resonance imaging” (42,43,44,45,46,47).
Magnetism and Magnetic Units
The official metric unit for magnetic field strength in the units of the Système International (SI) is the tesla, and the official abbreviation for this unit is capital “T” (48,49). In addition to being officially sanctioned, the tesla is of a convenient size to specify the strengths of main magnetic fields used in MRI. However, another unit of magnetic field strength, the gauss (abbreviated “G”), although not an official SI unit, has had a long historical usage and is still widely used by physicists and chemists; it is of a convenient size to describe the smaller fields (e.g., gradient fields) associated with MR scanners. The two units have an easy-to-remember relationship: 1 T is equivalent to 10,000 G. In this chapter, we use whichever of these units are more convenient for the magnetic field under discussion.
Magnetism was originally noticed more than 2,500 years ago as a property of certain rocks and minerals such as lodestone, which is now known to be the iron oxide (Fe3O4) and is called magnetite by geologists. Based on experiments with lodestones performed around the year 1600, the English physician William Gilbert proposed that Earth is a huge magnet and that it produces what would now be described as a dipole field. Although they are generally unaware of it, everyone on Earth is continually immersed in this field.
In 1820, the Danish physicist and chemist Hans Christian Oersted discovered that magnetic fields are also produced by electric currents flowing in wires. By the 1920s, modern atomic theory and quantum mechanics were developed to the point that the magnetic properties of lodestone and other magnetic materials could be described in terms of the motion of electrons within them. To achieve agreement with experiment, it was necessary to consider in addition to the orbital and translational motion of the electrons a further degree of freedom known as electron spin, which can be roughly visualized as a spinning motion of the electrons around their axes. In the presence of a steady magnetic field, the electron spins undergo a motion called precession that is analogous to the motion of a spinning top in a gravitational field. The spin axis precesses around the direction of the field at a fixed rate that is proportional to the strength of the applied field. This precessional motion repeats at a rate known as the Larmor frequency. All of the magnetic properties of materials and electric circuits can thus be explained in terms of the motion of electrons.
Also, in the 1920s, certain subtle effects in atomic spectra were noted that could be explained by associating a spin degree of freedom with some atomic nuclei. This led to the development of the field of nuclear magnetism (50,51). The behavior of nuclear spins is similar to that of electron spins, but the magnitudes of their effects are very much smaller than those of electrons, and it was initially doubted whether direct macroscopic effects of nuclear magnetism would ever be observed. As mentioned previously, in 1936, Gorter proposed a resonance method as a possible means of detecting nuclear magnetic effects. His protocol, called NMR, consisted in exposing nuclear spins to a strong magnetic field for a time long enough to align many of them with this field and then exciting them by use of an RF field at right angles to the initial field. This methodology was eventually shown to work and is the basic working principle for modern MRI devices.
Nuclei of many of the chemical elements can be studied using NMR, but far and away the most important for medical applications is the simplest atomic nucleus, the proton, which is the nucleus of atomic hydrogen. The magnetic moment of the proton is 658 times smaller than that of the electron, and this largely accounts for the difficulty in demonstrating macroscopic proton nuclear magnetism without the use of specialized equipment. The Larmor frequency for electrons and for all nuclear spins is linearly proportional to the applied magnetic field strength. For unshielded protons at 1 T, it is 42.577482 MHz. Whole-body clinical MRI studies have now been reported over at least the range from 0.02 to 9.4 T (200 to 94,000 G). This corresponds to a variation in Larmor frequency from 0.852 to 400 MHz. Therefore, the range of field strengths useful for medical imaging has been demonstrated to vary by a factor of at least 470. By using small magnets, much stronger fields can be obtained, and MRI studies of small animals and pathology specimens have been reported up to fields of at least 21.1 T, corresponding to a frequency of almost 900 MHz.
The human body contains an enormous number of hydrogen atoms, particularly in its water and lipid components. The proton spins can be manipulated by applied magnetic fields generated by electric currents in coils located outside the body, and signals produced by the motion (precession) of these spins can be detected using receiver coils that are also located outside the body. It turns out that, at the levels required for MRI, these applied electromagnetic fields have very little measurable influence on human tissues other than those on the nuclear spins. Some exceptions that occur at very powerful field levels or in the presence of magnetic foreign bodies will be discussed later. The remarkable fact that the elaborate electromagnetic activity associated with MRI couples strongly to the proton spins throughout the body, while the other components of human tissues are to a high degree transparent to these fields is the physical basis of the highly noninvasive character of this imaging modality.
The strength of Earth’s magnetic field is roughly 1/2 G, or 0.00005 T, and the proton Larmor frequency in Earth’s magnetic field is only about 2.1 kHz. The degree of nuclear spin magnetization in such a feeble field is far too small to result in an observable NMR signal without the use of special preparation techniques, and, as a result, Earth’s field is not useful in clinical MRI. The NMR signal is proportional to both the degree of spin magnetization and the rate of Larmor precession. In turn, these factors are both directly proportional to the magnetic field strength, Bo. Therefore, the NMR signal strength increases as the square of Bo. However, patient-generated electrical noise increases approximately linearly with frequency. This results in a signal-to-noise ratio (SNR) that increases, in strong fields, roughly in direct proportion to Bo, and this gives an SNR advantage to higher field scanners. This advantage must be balanced, of course, with other factors, such as cost and size, in deciding on the Bo field to use for a given scanner.
Types of Magnetic Field Coils
MRI requires the application to a human patient of several strong and carefully crafted magnetic fields that are controlled and varied as precisely defined functions of space and time within the patient. Magnetic fields are commonly produced by electric currents in metallic conductors. Very intense magnetic fields are often produced by winding long metal wires into dense coil structures. When this is done, the field produced by the current in each turn is superimposed on those of the other turns, and a strong field can be produced from a relatively small current. For these historical reasons, the current-carrying field sources in MRI are usually referred to as coils, although, in many instances, other conductor shapes (such as conductor patterns etched in sheets of copper) are used rather than wire coils. Every MR scanner contains several sets of coils that serve as sources of the different magnetic fields that are used to manipulate the magnetic spins within the patient. Scanners also contain receiver coils and associated electronics to detect and amplify the very weak RF fields that originate within the patients but that can be detected outside of them once appropriate patterns of spin orientation have been prepared.
In a somewhat simplified view, a scanner requires the use of three types of coils. The first set of coils produces an intense uniform (i.e., homogeneous) main field that is constant in time and is used to align (or magnetize) the spins within the patient. This field also drives the Larmor precession of the spins once the transmitter coil has excited them. Second, there is a set of three gradient coils. Each of these coils produces a gradient field that can be pulsed on and off during the scan to give the field (and, therefore, the precessional motion) a slightly different time dependence at different positions within the patient. Finally, there is a spatially uniform RF magnetic field oscillating at or near the Larmor frequency of the protons and which is pulsed on and off to produce repetitive excitation of the spins within the patient.
The main magnetic field needs to be very uniform in terms of its strength and direction. It defines a direction in space that is conventionally referred to as the z direction. The z component of the main field at the center of the magnet is designated Bo and is so strong that it completely dominates the effects of the small transverse fields (Bx and By) that are inevitably produced by the main magnet and the gradient coils. The transverse Bx and By fields are, therefore, usually inconsequential in determining the MR signal, and we will not be concerned with them any further. As discussed later, the z axis usually runs along the cylindrical axis of the main magnet and in the direction from the patient’s head to the feet (superior to inferior). Any variation in the longitudinal field, Bz, from its central value, Bo, has a significant effect on the motion of the spin system and, therefore, also on the MRI process. Consequently, the design of the main field coils is chosen to minimize variations in the strength of the Bo field over the desired region of imaging.
Three separate gradient coils, one each for dBz/dx, dBz/dy, and dBz/dz, are used to produce controlled variations in Bz and, thereby, to permit slice selection and to encode position-dependent information in the MR signal. Ideally, the gradient coils produce longitudinal Bz fields that are precisely zero at the center of the scanner and that vary in a perfectly linear fashion over the region of imaging. Thus, we have three fields that can be independently specified, Bx = Gxx, By = Gyy, and Bz = Gzz. The parameters Gx, Gy, and Gz are referred to, respectively, as the x-, y-, and z-gradient strengths. They are usually specified in terms of tesla/meter or gauss/centimeter. It is important to realize that although each of the gradient coils individually produces a field in a specific Cartesian direction, a gradient field in any arbitrary direction in space can be generated by activating two or all three of the gradient coils, with the proper current levels, simultaneously. This makes it possible to select imaging planes in any one of the principal orientations—axial, sagittal, or coronal—and also in any oblique orientation. This flexibility to select any desired scan plane electronically and without moving the patient gives MRI one of its major advantages over computed tomography and other imaging modalities.
An additional coil (or coils), called the transmitter coil, carries currents at radiofrequencies at or near the Larmor frequency and is used to excite the spins so that they begin to precess in the main magnetic field and to provide the signal that is used to construct the image. This signal is then detected by a receiver coil that is also tuned to the Larmor frequency. The receiver coil may be the same coil as the transmitter, or it may be a separate structure. The RF excitation field is at right angles to the main magnetic field. Therefore, it is a Bx or By field or some combination of these two components. The components are of the general form Bx or By = B1 cos(ωt + φ), where the frequency, ω, is at or near the spin Larmor frequency. Ideally, the amplitude, B1, and the phase, φ, do not depend on position. Circularly polarized fields, produced by superimposing Bx and By fields with a 90-degree phase difference, are often used because they offer efficiency improvements over linearly polarized fields in both the transmit and receive modes.
To summarize, an ideal scanner produces the following magnetic fields over the entire region to be imaged. There is an intense, perfectly uniform main field, Bz = Bo, which is constant in time and space. There are three gradient fields each varying linearly with position and of the form Bz = Guu with u = x, y, or z and, respectively, u = x, y, or z. Finally, there is an oscillating RF field in the direction perpendicular to z that is completely uniform in space. It is seen that a minimum of five separate coils—one main field coil, three gradient coils, and one RF coil, each producing a distinct field pattern—are required to construct an idealized MR scanner. The gradient and RF coils are independently activated under computer control to produce a desired pattern of time-dependent spin excitation and precession. The pattern of these gradient and RF excitations is called a pulse sequence (38).
In addition to the three major coil types just discussed, MR scanners may contain a set of several additional static field coils called shim coils. These are specially patterned coils designed to correct inhomogeneities that may be present in the Bo field that result from manufacturing tolerances in the main field magnet or from other sources of field distortion. The currents in these shim coils are normally set to optimum values during scanner installation and are reset from time to time as part of the periodic scanner maintenance process.
Main Field Magnets
The main field magnets are the key component of MR scanners, and more than any other factor they determine the appearance, cost, and capabilities of these devices. The most important characteristics of these magnets are:
the magnetic field strength at the center of the imaging region;
the uniformity of the magnetic field over the desired field of view;
the temporal stability of the magnetic field for the duration of the image acquisition;
the size of the magnet must be large enough to produce this strong, stable, uniform magnetic field over large regions of the adult human body; and
the ability to keep the patient safe and comfortable for the duration of the study.
There are also a number of secondary features that affect the suitability of a magnet for operation in a specific environment. These features include:
the weight and external dimensions (height and length) of the magnet;
the magnitude and the spatial extent of the fringing magnetic field surrounding the magnet; and
the cryogenic requirements and the quench resistance for superconducting magnets.
There are several basic options available for the design and construction of MRI main magnets:
Permanent magnets made from “hard” magnetic materials. These magnets do not require any external power source.
Electromagnets (iron-core magnets) made from “soft” magnetic materials and energized by electric currents flowing in wires wound around a portion of the magnet.
Resistive magnets (sometimes called air-core magnets) that utilize electric currents in metallic wires or tapes (usually copper or aluminum) wound into a series of circular coils arranged along a cylinder surrounding the patient.
Superconducting magnets using a coil geometry similar to that of resistive magnets but which utilize zero-resistance superconducting metal wires (a niobium–titanium alloy cooled to near absolute zero) as the current conductors. These magnets have an important property in common with permanent magnets in that, unlike electromagnets and resistive magnets, they require no external power source once energized.
Hybrid magnets that combine features of more than one of these basic types. For example, a magnet might include both a set of permanent magnets and a set of superconducting coils to achieve a stronger field than could be produced by either set acting alone.
Magnets of all these types have been used successfully for human MRI imaging. However, because of their ability to produce very strong and highly uniform magnetic fields and to maintain a high degree of temporal stability, cylindrical superconducting magnets have dominated the field of MRI since the mid-1980s. Nonetheless, scanners based on other types of magnets are used in various niche applications. These include low-cost systems and scanners restricted to limb imaging. Also, so-called open systems, using a hybrid structure containing both superconducting and permanent magnets, can provide advantages when imaging claustrophobic or extremely heavy patients (Fig. 1.1). Because of their dominant role in modern, high-performance neuroimaging, the rest of this chapter will discuss only superconducting magnets.
Superconducting Materials for MRI Magnets
Superconductivity was discovered as an exotic property of certain metals at very low temperatures in 1911 and subsequently became a major research topic of theoretical and experimental solid-state scientists. The most remarkable property of these materials is that they have absolutely no electrical resistance when cooled below their superconducting transition temperatures. However, this temperature is so low that liquid helium is required to cool the metals to the point where superconductivity appears. It is natural to try to build powerful magnets from any material with zero electrical resistance. Unfortunately, for the superconductors known before about 1950 (the type I superconductors such as lead, tin, and mercury), once the current density exceeds a rather low critical value, the superconductivity vanishes and the resistance returns. Magnets made from these materials are, therefore, limited to producing disappointingly weak magnetic fields—a small fraction of a tesla at most. This left superconductivity as a fascinating scientific phenomenon but with very little commercial significance. However, the discovery of a new class of high-field superconducting alloys (type II superconductors) in the 1950s opened the way to producing much more powerful magnets. After a great deal of metallurgical experimentation on hundreds or thousands of materials carried out in many laboratories, Berlincourt and Hake announced in 1962 (52) that an alloy of niobium and titanium could carry superconducting currents at densities 30 times the current densities that were feasible in copper-wound resistive magnets. Moreover, it turned out that the Nb–Ti alloys had the very desirable metallurgical property of high ductility and could be readily formed into superconducting wires thousands of meters long. These wires could then be wound into superconducting coils capable of producing magnetic fields of several tesla. It has been emphasized that, although many materials with superior superconducting properties (superconducting transition temperature and critical current density) than Nb–Ti have been found, these materials have generally been brittle, expensive, and hard to fabricate into coils (53). Thus, the Nb–Ti alloys have dominated the superconducting magnet business to the present time because of its ductility which provides ease of fabrication and suitability for bulk production of reliable, cost-effective superconducting wire. However, except for customized magnets designed for research purposes, there was no large-scale commercial application of superconductivity, including these type II materials, prior to the advent of whole-body MRI in the early 1980s.
Superconducting Magnets
As the great clinical potential of MRI became appreciated during the early 1980s reservations regarding the technical and cost challenges associated with whole-body superconducting magnets rapidly gave way to enthusiasm for the advantages they offered. Superconducting magnets are designed to achieve a prescribed maximum field strength and, in principle, can be operated to produce any field strength up to this design value. In practice, however, they are almost always operated at a fixed, constant field, near the upper limit of their capabilities. Because of the superconducting properties of the magnet coils, once the desired field strength is reached and the superconducting circuit is closed, the power supply can be disconnected, and the magnet
will operate in the persistent mode. That is, the full field strength can be maintained, without additional power input, for months or years. Typical performance specifications for these magnets are that, at the magnet center, the field is spatially uniform (i.e., homogeneous) to better than 10 parts per million (ppm) over a sphere 40 to 50 cm in diameter and that the drift of the field (i.e., temporal stability) is less than 0.1 ppm per hour. Generally speaking, the cost and manufacturing difficulty of building these magnets increase rapidly with the prescribed field strength and the diameter of the warm bore (the central opening where the patient is located during imaging). Although largely out of sight, the interior components of a superconducting whole-body magnet are remarkable high-technology devices operating with very tight tolerances, at extremely low temperatures and supporting enormous magnetic forces (54,55,56).
will operate in the persistent mode. That is, the full field strength can be maintained, without additional power input, for months or years. Typical performance specifications for these magnets are that, at the magnet center, the field is spatially uniform (i.e., homogeneous) to better than 10 parts per million (ppm) over a sphere 40 to 50 cm in diameter and that the drift of the field (i.e., temporal stability) is less than 0.1 ppm per hour. Generally speaking, the cost and manufacturing difficulty of building these magnets increase rapidly with the prescribed field strength and the diameter of the warm bore (the central opening where the patient is located during imaging). Although largely out of sight, the interior components of a superconducting whole-body magnet are remarkable high-technology devices operating with very tight tolerances, at extremely low temperatures and supporting enormous magnetic forces (54,55,56).
As mentioned above, whole-body superconducting magnets have been used in MRI clinical applications and research over a wide range of field strengths. However, for technical and historical reasons, the majority of superconducting whole-body magnets placed into clinical practice between the mid-1980s and the present operate at 1.5 T. Since approximately the year 2000 there has been a significant increase in the clinical use of whole-body scanners operating at 3 T. This is done to take advantage of the improved SNRs and improved resolution available at higher field strengths. Still more recently, whole-body systems operating at 7 T have been placed in a number of research centers. At the present time, there are roughly 25,000 1.5-T and 5,000 whole-body 3-T systems in place worldwide. 1.5-T magnets still account for about three-fourths of the new installations but the fraction of 3-T systems in new installations is increasing with time.
Figure 1.2 is a cutaway drawing illustrating many of the features of modern MRI superconducting magnets. In this example, the field is produced by an intense current flowing through six coils that are connected in series. The structure that contains the cryogenically cooled coils is called the cryostat. A typical superconducting magnet has a total of about 5 to 7 main coils on coil forms of about 0.65 m radius surrounded by two shielding counter coils on a larger radius. The total length of the superconducting wire is on the order of 120 km or 75 miles. This total length of wire must be constructed without any interruption of its superconducting properties in order to achieve persistent operation and adequate temporal stability but the total wire length wire is made up of 10 to 20 shorter segments. The technical problem of joining shorter segments of wire while maintaining the superconducting properties at the linkages was one of the major challenges in the development of superconducting magnets. The total weight of the mass at helium temperature of a modern 3-T magnet (superconducting wire and its supporting structures) is on the order of 9,000 lb. The warm bore opening of the main magnet is normally in the range from 85 to 100 cm. After allowance is made to accommodate the gradient coils and RF transmit/receive coils, the diameter of the space available for the patient and the patient support system is on the order of 60 to 70 cm.
The superconducting coils are immersed in a bath of liquid helium at 4.2 K or somewhat colder to cool the niobium–titanium alloy well below its superconducting transition temperature, which is about 9.2 K. In the original superconducting magnet designs, the transition from liquid helium temperatures to room temperature (298 K) was made through an intermediate liquid nitrogen stage at 77 K. With improved technology such as the use of cryogenic refrigerators, it is now no longer necessary to include an intermediate stage at liquid nitrogen temperature. Nonetheless, there is an inexorable boiling of the cryogenic liquids due to thermal energy
entering the helium bath from the outside. This necessitates periodic refilling of the cryogenic fluids. These refills were originally required every few weeks. Now it is possible to go for several months, and even longer in many cases, between helium refills. Figures 1.3 and 1.4 show, respectively, the appearance of a 3-T and a 7-T magnet.
entering the helium bath from the outside. This necessitates periodic refilling of the cryogenic fluids. These refills were originally required every few weeks. Now it is possible to go for several months, and even longer in many cases, between helium refills. Figures 1.3 and 1.4 show, respectively, the appearance of a 3-T and a 7-T magnet.
To maintain the superconducting state the conductor must be kept below its transition temperature. If even a small region of the wire is heated above this temperature, it begins to dissipate heat and the temperature increases still further. The result can be a self-propagating process leading to a magnet quench, wherein the entire stored energy in the magnetic field is converted into heat and the metal returns to its normal resistive state. This raises the temperature of the liquid helium above its boiling point and it quickly evaporates as the magnetic field collapses. Magnets are designed to sense the onset of a quench and to rapidly activate heaters that spread the energy deposition throughout the coils. Otherwise, the deposition of the total stored energy in a single location could melt the wire locally and destroy the magnet. In a controlled quench, the field will decrease from its initial value to nearly zero in about 1 minute. Provision must be made to permit the safe removal of the helium gas that is produced during a quench. This gas is very cold and direct contact with it should be avoided. Also by displacing room oxygen, a quench, if the gas is not properly vented, represents a suffocation risk to people nearby. All the wires in a superconducting magnet are subject to intense forces that can cause slight movement and local frictional heating that may be sufficient to initiate a quench. This possibility is minimized by a process called training. This is one of the final steps in magnet manufacture and involves cycling the magnet to fields somewhat above its planned operating field to assure stable operation at the rated field. Quenches are also possible if a cryostat vacuum fails or if necessary refills of the cryogenic liquids are not made on time. Superconducting magnets are supplied with an emergency switch system that permits a deliberate, but nondestructive, quench in the event that someone has become trapped against or inside the magnet by a ferromagnetic object, such as an oxygen bottle.
![]() FIGURE 1.4 High-field research system. A 7-T research magnet with patient table is shown. This magnet is roughly twice the length of the 3-T magnet (Fig. 1.3) because a compensated solenoid winding geometry is used here rather than the discrete coil approach commonly used at lower field strengths. The solenoidal coil design requires the use of more superconducting material than does the discrete coil design. However, it substantially reduces the maximum field strength at the superconducting coils. (Courtesy of General Electric.) |
In recent years, both the cost and availability of liquid helium have become somewhat problematic. Helium is generally collected as part of natural gas extraction and is produced from radioactive decay of neutron-rich materials like uranium. Since helium is much lighter than nitrogen (the predominant gas in the Earth’s atmosphere) and is generally nonreactive chemically, once released as a gas it floats away and is lost. Three technologies are generally considered important candidates for reducing helium consumption. First, “zero boiloff” magnets incorporate recondensing compressors (often called “cold heads”) that rely on the Gifford–McMahon cycle to extract heat from the helium gas and recondense it to a liquid, maintaining the low temperature for the superconductor. Human-sized magnets with field strengths up to 7 T have been produced using recondensing compressors. Second, “cryogenless” magnets rely on a large mass of metal that, once cold, can maintain a low temperature. While these systems can operate with very little helium, care must be taken that, should a quench occur, heat will be extracted from the superconducting wire quickly enough to prevent damage to the wire. While few magnets to date have been built using this technology, it is likely to become an important technology particularly at clinical field strengths (1.5 and 3 T). Third, more exotic superconducting materials, such as MgB2, are able to maintain a superconducting state at a higher temperature, permitting the use of more readily available cryogens. These materials are generally less ductile and far more expensive than Nb–Ti alloys, limiting their applicability for the foreseeable future.
Shim Coils
There are inevitable imperfections in the coil locations within a manufactured magnet. These imperfections lead to the presence of error fields which reduce the uniformity of the field within the field of view (FOV) below what it would have been if the coils could have been wound and positioned exactly as in the ideal design.
Various shimming techniques are used to tweak the final magnetic field to correct for these inevitable manufacturing errors and for additional errors that result from the magnetization of magnetic materials, such as building girders, in the
vicinity of the magnet. Passive shimming, somewhat analogous to tuning a piano, is accomplished by positioning small ferromagnetic strips at specific locations within slots around the bore of the magnet (Fig. 1.2) to counteract field inhomogeneities once they have been mapped and characterized (57).
vicinity of the magnet. Passive shimming, somewhat analogous to tuning a piano, is accomplished by positioning small ferromagnetic strips at specific locations within slots around the bore of the magnet (Fig. 1.2) to counteract field inhomogeneities once they have been mapped and characterized (57).
Active shimming is an additional method for improving magnet homogeneity. This technique makes use of sets of specially designed coils placed within the magnet each of which is designed with a specific symmetry to produce a field closely approximating an idealized field referred to mathematically as a spherical harmonic. If superconducting shim coils are used as shown in Figure 1.2, they are located, like the main coils, within the cryostat and are maintained at liquid helium temperature. To make it possible to change the current in superconducting shim coils, it is necessary to provide current leads to an external power supply. These leads can be switched from one shim coil to another and a set of currents can be built up in the array of coils to cancel out each of the harmonic fields for which a shim coil has been provided. Superconducting switches are used to restore each coil to the persistent mode once the power supply has brought the shim current to the desired value. An alternative or supplement to the use of superconducting shim coils is to provide a set of resistive shim coils which can be located within the room temperature bore of the magnet adjacent to the gradient coils (not shown in Figure 1.2). Each of these coils also is designed to produce primarily a single harmonic field but, in this case, each coil is permanently connected to its own power supply. This makes it relatively easy to reset the resistive shim coils as necessary.
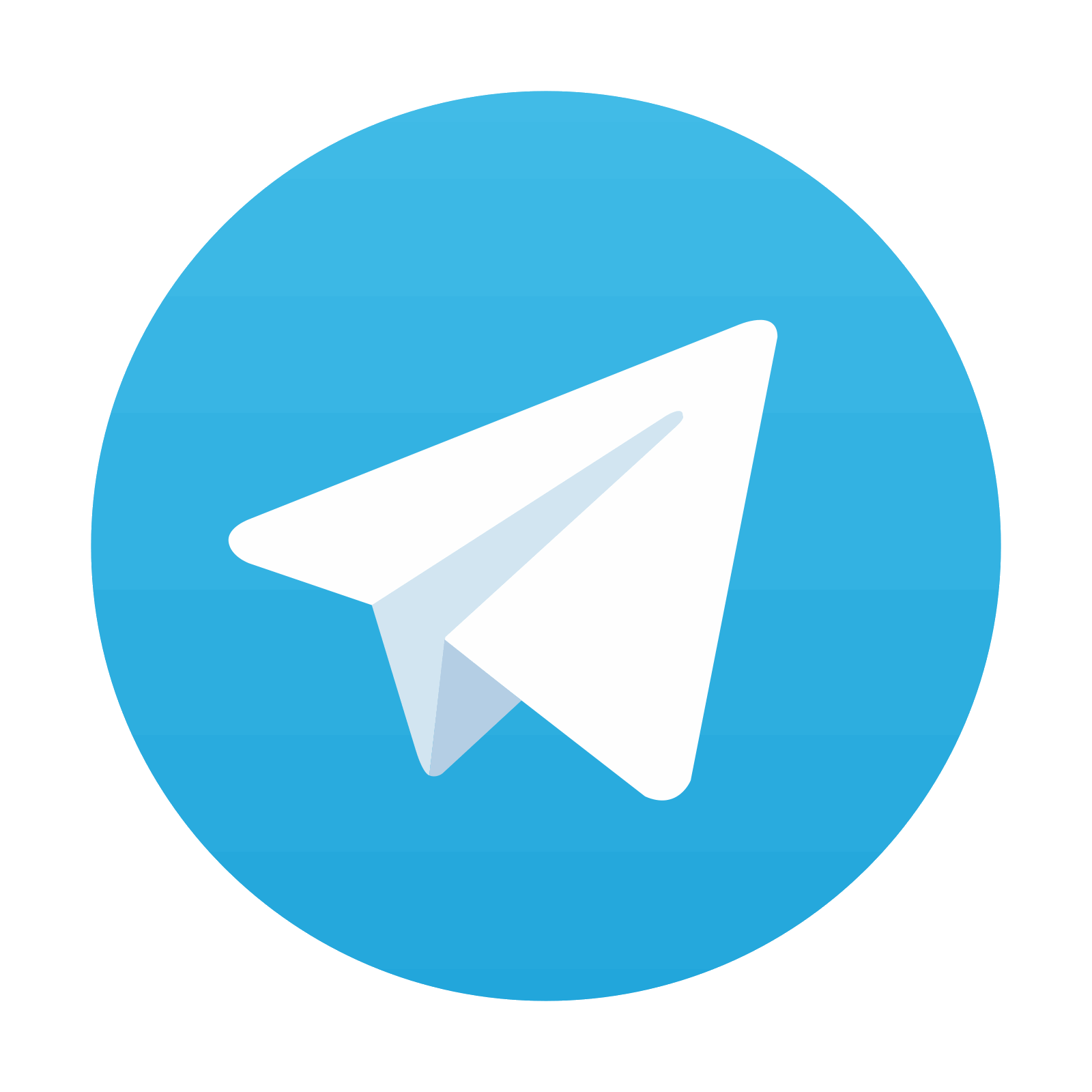
Stay updated, free articles. Join our Telegram channel
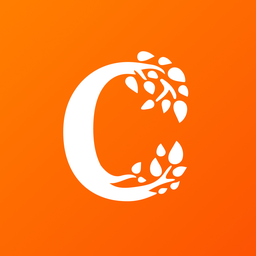
Full access? Get Clinical Tree
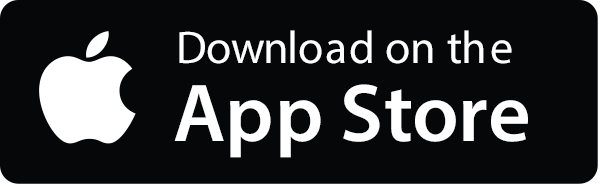
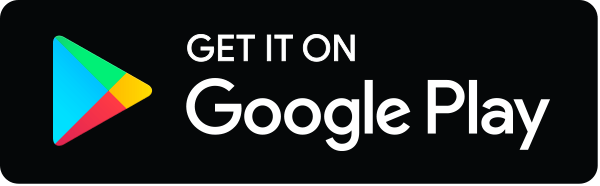