Fig. 1
Fluorescence excitation and emission from quantum dots. (a) Energy diagrams of a bulk semiconductor and a quantum dot. (b) Fluorescence spectra measured from different types of quantum dots. Reprinted from Ref. [12], copyright (2014), with permission from Elsevier. (c) Fluorescence resonance energy transfer (FRET). When the distance between the donor and the acceptor is close enough, FRET occurs and emission from the acceptor is observed
The molecular orbitals in the valence band are bonding orbitals, which are full. The orbitals in the conduction band are antibonding orbitals and are vacant. The energy level difference between the highest occupied molecular orbital (HOMO , the top of valence band) and the lowest unoccupied molecular orbital (LUMO, bottom of conduction band) defines the band gap of the colloidal QD. When an electron in HOMO is excited, it moves to an orbital in the conduction band, leaving a hole in HOMO (Fig. 1a left). The electron eventually goes back to HOMO and fluorescence is observed as a result of the transition, which can be also considered as the recombination of the electron and the hole (Fig. 1a right). The emission wavelength is defined by the band gap, or the difference between the LUMO and the HOMO. The band gap is dependent on the size of QDs. Smaller quantum dots have larger band gaps because the quantum confinement effect is stronger. Because of this size effect, the emission wavelengths of QDs can be tailored by controlling the diameters of the particles. The emission wavelength becomes shorter for smaller particles because the emission energy is larger. Emission spectra measured from different types of colloidal QDs (Fig. 1b).
For colloidal quantum dots, the mechanism of fluorescence emission is defined by this simple structure of the energy levels, and less affected by the excitation wavelengths. Colloidal QDs can be excited by any wavelength shorter than the emission wavelengths. Typically, a UV light will excite all types of colloidal QDs with visible emission wavelengths. On the other hand, most organic fluorescent materials follow the mirror image rule, where and absorption spectrum is similar to a mirror image of the emission spectrum. An excitation light for a certain fluorescent dye may not excite other fluorescent materials. This difference is important in designing fluorescent optical systems using either colloidal QDs or organic fluorescent materials.
QDs with a core–shell structure is often used to obtain stability. One example is QDs with a CdSe core capped with a 1–2 monolayers of ZnS. The shell is used to passivate the core surface and protect it from surrounding solutions. The structure prevents crystal defects which lead to unwanted electron or hole traps, and substantially improves the photoluminescence intensity and stability [5]. Colloidal QDs are typically prepared in organic solvent. They are usually capped with hydrophilic surface ligands when used in an aqueous solution or further chemical attachment of functional biomolecules is needed.
2.2 Quantum Dots as a Fluorescence Marker
QDs are alternatives to organic fluorescent dyes for biological imaging and sensing. They can be functionalized with biomolecules to label specific biomaterials. A notable early study for immunofluorescence imaging with quantum dots used breast cancer marker Her2 to selectively stain fixed and live cancer cells [6].
Characteristics of organic dyes and QDs have been compared in [7], with important differences for hyperspectral imaging being summarized in Table 1. Along with the tunable emission wavelengths, the photo stability is also a significant advantage of QDs. For example in [6], the nuclei and microtubules of mouse 3 T3 fibroblast cells are stained with either colloidal QDs or the organic dye Alexa 488 with fluorescence intensities being compared by taking time lapse images. After 180 s, the fluorescence intensity of QDs were almost unchanged, while the normalized intensity of the 488 dye became about 50 % because of the photobleaching induced by continuous fluorescence excitation. One of the notable difficulties associated with the use of QDs as a fluorescent marker is the size. QDs behave as nano-colloids rather than as molecules, and the intracellular delivery of QDs is still challenging [7]. Methodologies to deliver QDs into cells for intracellular imaging are behind those developed for organic dyes.
Property | Organic dye | QD |
---|---|---|
Absorption spectra | Discrete. FWHM 35–100 nm | Steady increase toward UV with a single peak |
Emission spectra | Asymmetric, often with a tail to long wavelength side | Symmetric Gaussian distribution. FWHM 30–90 nm |
Quantum yield | 0.5–1.0 (visible), 0.05–0.25 (NIR) | 0.1–0.8 (visible), 0.2–0.7 (NIR) |
Size | ~0.5 nm molecule | 6–60 nm in hydrodynamic diameter |
2.3 Fluorescence Resonance Energy Transfer (FRET)
QDs can be used as a light source for fluorescence excitation. One of the applications where QDs are used as a excitation source is sensors based on fluorescence resonance energy transfer (FRET). FRET is a nonradiative energy transfer between the excitation source (donor) and the fluorescence molecule (acceptor). FRET occurs when donor-to-acceptor separation is at distances typically smaller than 10 nm (Fig. 1c). Because of this nanoscale sensitivity to distance, FRET can be used to detect changes in distances at the nanometer scale. Medintz et al. developed a chemical sensor that utilized FRET to detect changes in the distances between molecules [8]. The sensor molecules containing a QD, fluorescent molecules and maltose-binding molecules that bind the QD and the fluorescent molecules. Because the distance between QD and the fluorescent molecules are closer than the FRET distances, the emission from the QD is quenched by the fluorescent molecules and not observed in the initial status. When maltose is added, a maltose-binding protein takes maltose molecules and releases fluorescent molecules from the QD sensor molecules, resulting in the increase of QD emission. Zhang et al. developed a single QD-based DNA sensor [9]. A QD molecule is linked to DNA probes to capture DNA targets. When a target strand is sandwiched by a dye-labeled reporter strand (acceptor) and the DNA probes on the QD (donor), molecules form a pair of FRET donor and acceptor, and the excited reporter fluorescent dye emits fluorescence to be observed. In [10], Hohng et al. developed a QD-dye molecule (cy5) pair using a molecular junction composed of four DNA double helices. They measured FRET signals induced by conformational changes of individual molecules.
3 Quantum Dots as a Light Source for Hyperspectral Microscopy
In this section, we describe our recent studies that utilized colloidal QDs as light sources for microscopic spectroscopy. In [11] and [12], we used photo-excited QD light sources for absorption spectroscopy and fluorescence spectroscopy, respectively. In the following Sects. 4.1 and 4.2, we describe our unique efforts to use electrically excited colloidal QDs in microelectromechanical systems (MEMS)-based microscopes.
3.1 Colloidal QDs for Absorption Microscopy
The tunable emission wavelengths described in Sect. 2 make colloidal QDs an excellent light source for hyperspectral microscopy. We have used an array of QD light sources to construct a uniform, solid state swept light source that covers the visible–IR wavelength range. In the systems we reported in [11], QDs are excited by UV lights from LEDs. Figure 2a shows a photograph of QDs packed in a polydimethylsiloxane (PDMS) enclosure that is replicated from a plastic mold fabricated by rapid 3D printing. There is an array of miniature wells, each of which contains a single color of QDs. Figure 2b illustrates the design of the light source. The central concave-shaped circular part with multiple dimples transmits and scatters emission from the QDs. Digital on–off control of arrayed LEDs selectively excites QDs with desired color. UV-LEDs with the emission peak at 380 nm were used. Emission wavelengths ranging from 520 to 800 nm were obtained by 8 colors of CdSe/ZnS and CdTe/ZnS colloidal quantum dots. A top plate consisting of a diffuser and a 400 nm long-pass filter is placed on top of the concave surface to cut off the excitation UV lights. Typical hyperspectral microscopes employ mechanically controlled color wheels or mechanical stages that make the entire system more expensive. The QD-based swept light source does not contain any moving part, and is simply controlled by LabVIEW™ based digital switches to turn on/off the UVLEDs. It can also create any linear combination of the eight colors by changing duties ratios of excitation UVLEDs.
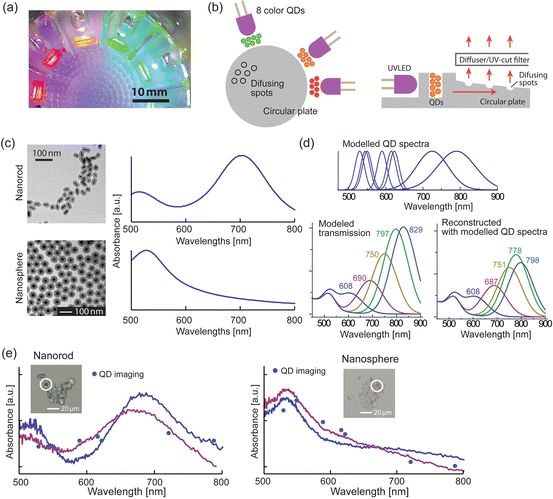
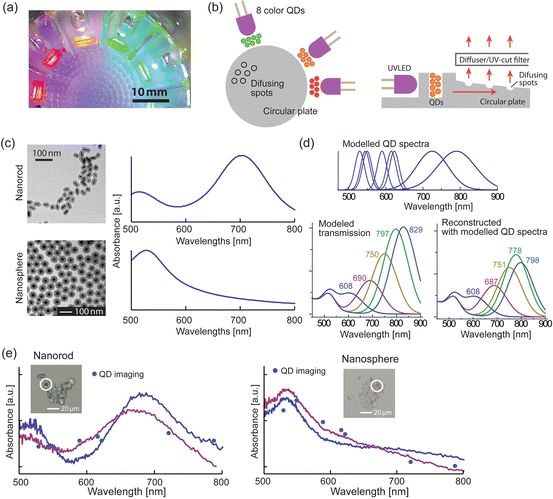
Fig. 2
Absorption spectroscopy with the QD swept light source. (a) Photograph of QD-based swept light source (b) Schematic of light source. Emission of 520–800 nm can be chosen by digital on–off control of the excitation UVLEDs. (c) TEM micrographs along with optical transmission spectra of silica coated (top) nanorods and (bottom) nanospheres. (d) Using the modeled nonuniformly distributed QD spectra (top), transmission spectra of different types of gold nanorods (bottom left) were reconstructed (bottom right). (e) Absorption spectra measured from the reference hyperspectral images and the QD swept light source for macrophage cells stained (left) with nanorods and (right) with nanospheres
3.1.1 Gold Nanorods and Nanospheres as Contrast Agents
Metal nanoparticles have size and shape-dependent characteristic absorption spectra due to the effect of localized surface plasmon resonance, and they can be used in hyperspectral absorption spectroscopy [13]. In the case of gold nanorods, one of the two peaks is dependent on the aspect ratio (length-to-diameter ratio), and can be tuned between ~600 nm and ~800 nm by controlled chemical synthesis [14]. Although the mechanisms are different, the tunable absorption peaks of nanorods are complementary to the emission peaks of colloidal QDs. The use of gold nanoparticles as optical markers gives an edge to the hyperspectral microscopy based on the QD swept light source.
One problem associated with the gold nanoparticles used for optical labeling is plasmon coupling. When two metal particles are close to each other, broadening of optical spectra is observed due to the electromagnetic interaction between them. For hyperspectral microscopy, it is crucial to reduce optical coupling and keep original optical properties of nanorods. We used gold nanoparticles that are coated by silica-shells, which successfully reduced plasmon coupling [15]. Figure 2c shows TEM photographs of gold nanorods and nanospheres (20 nm diameter) we used for the measurement. The transmission spectra of nanorods and nanospheres suspended in buffer solutions are also shown in Fig. 2c. The nanospheres were synthesized via citrate reduction of chloroauric acid. The narnorods were synthesized using a reaction described by Jana et al. [16] and Nikoobakht et al. [14].
3.1.2 Theoretical Analysis
We first studied the spectral resolution of the proposed system based on a theoretical analysis. In practice, the emission peak of colloidal QDs may be shifted from the nominal or designed value by about ±5 nm. In the model spectra of QD emission shown in Fig. 2d top, we incorporated errors we found in commercially available colloidal quantum dots (Life Technologies QDot®). Figure 2d bottom left shows modeled absorption curves of nanorods built by fitting a linear combination of Gaussian functions to the curves shown in Nikoobakht et al. [8]. Each curve is built from a fixed peak at 520 nm and an additional peak ranging from 608 to 829 nm. We simulated the hyperspectral measurement in the following way: Transmitted intensities of each color of QDs were calculated as a product of the emission spectrum and the absorption spectrum. For each type of nanorod suspension, transmitted intensities of eight colors of QDs were calculated. Using the 8 values and the QD spectra, the transmission spectrum of the nanorod can be reconstructed by curve fitting. The curves reconstructed based on the modeled QD spectra is shown in Fig. 2d bottom right. The results suggest that even with errors of about 5°, the swept light source can successfully resolve five different types of nanorods.
3.1.3 Experimental Results
Hyperspectral transmission imaging was conducted by using macrophage cells labeled with nanorods and nanospheres. Macrophage cells were incubated in media containing nanorods or nanospheres for 18 h and allowed to uptake nanoparticles. Cell suspensions are centrifuged and labeled cells are collected as pellets to be washed and fixed on a glass slide using Fluoromount. RGB color images of macrophage cells labeled with nanorods and nanospheres were taken with a 12-bit color mosaic CCD camera (SPOT Pursuit XS, Diagnostic Instruments) and shown in panels of Fig. 2e left and right, respectively. Nanorods stain cells blue, while nanospheres show pink colors. As a reference hyperspectral analysis, the same regions of the cells are imaged using a PARISS spectral imager (Lightform Inc.). The spectra measured from circled areas of the RGB images are shown in Fig. 2e left and right for the nanorod and nanosphere , respectively. The reference spectra were measured with a 20 × 0.5 NA objective and 100 W halogen light source. The lamp spectrum was obtained from the part of the microscope slide that did not have cells, and was used to normalize the hyperspectral images. For each image, spectra measured for two neighboring areas of 2 × 3 pixels are shown to indicate spatial deviation typically found in hyperspectral imaging. Curves show the same characteristic as the measurements from the particle suspensions in Fig. 2c.
The QD swept light source was then used to measured absorption characteristics of the same cell samples. Eight images illuminated by eight colors of the QDs were taken with a monochromatic cooled CCD (Pixis 400, Princeton instruments) with the wavelength sensitivity range of 450–1000 nm. The light intensity obtained from the part of the image that did not have cells was used as the denominator, and the absorbance for each LED was calculated for the spot indicated by the circle. The results were plotted as dots in Fig. 2e left and right for nanorods and nanospheres, respectively. Measurements with the QD light source fit within the range of deviation with the curves from the commercial hyperspectral microscope. QD imaging method clearly showed characteristic peaks of both nanorods and nanospheres.
We have shown that the use of QDs allows for low-cost hyperspectral imaging of biosamples with a simple standard microscope configuration. Proper choice of different gold nanoparticles, such as nanorods and nanospheres, will suitably fit absorption-based analysis using QD light multicolor sources. The use of QDs and gold nanoparticles demonstrated hyperspectral imaging for multi-biomarkers recognition at cellular level.
3.2 Colloidal QDs for Fluorescence Excitation
Figure 3a shows another type of QD light source we developed for fluorescence excitation. We adapted the microcontact printing technique reported in Ref. [17] to pattern CdSe/ZnS core–shell QDs onto a glass slide. An UV light (emission peak 365 nm) from a high-power LED (200 mW) is focused and introduced inside a glass slide which works as a wave guide. The patterned QDs are excited in an evanescent field on the surface of the glass slide induced by the total internal reflection of the transmitted UV light. When excited, the QDs work as an illumination source for microscopic imaging. The light source can be used in a standard microscope to add the functions of absorption and fluorescence imaging. The intensity of excitation observed from the microscope is negligible compared to the QD fluorescence, because the UV evanescent field on the glass slide decays in a sub-wavelength distance from the surface and does not transmit energy in the far-field. Emission wavelengths ranging from 520 to 650 nm were tested.
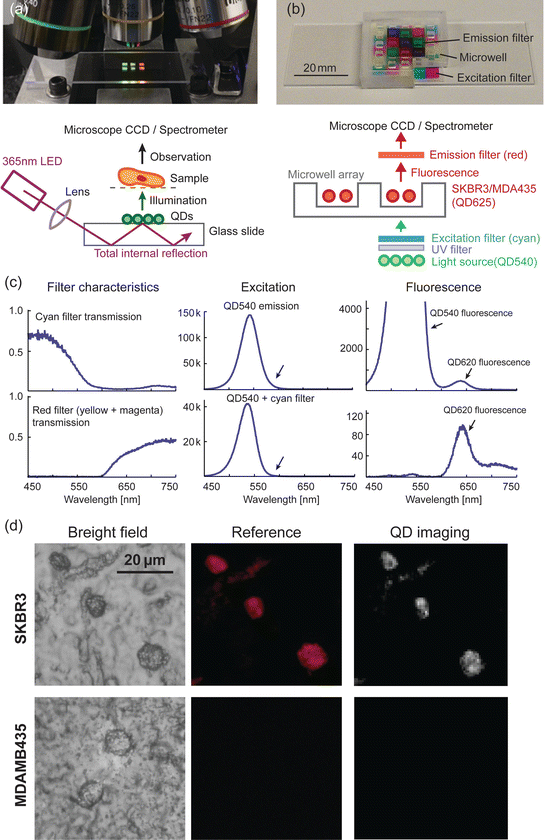
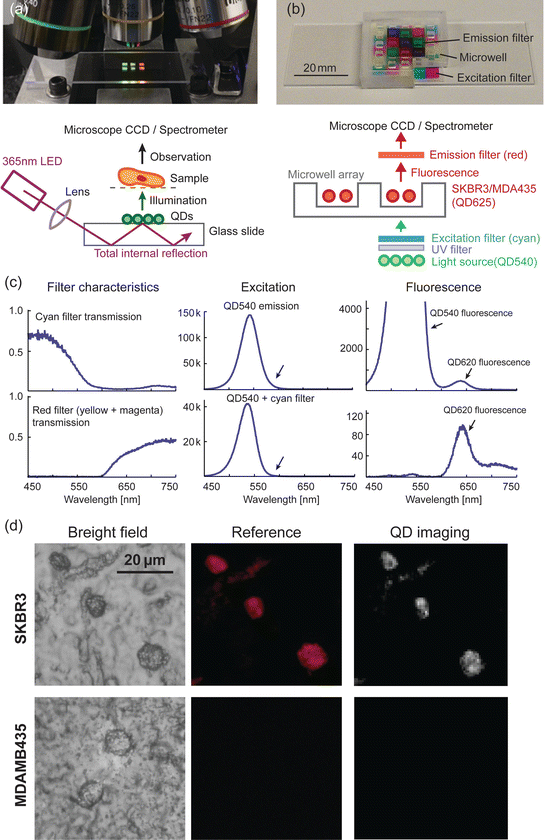
Fig. 3
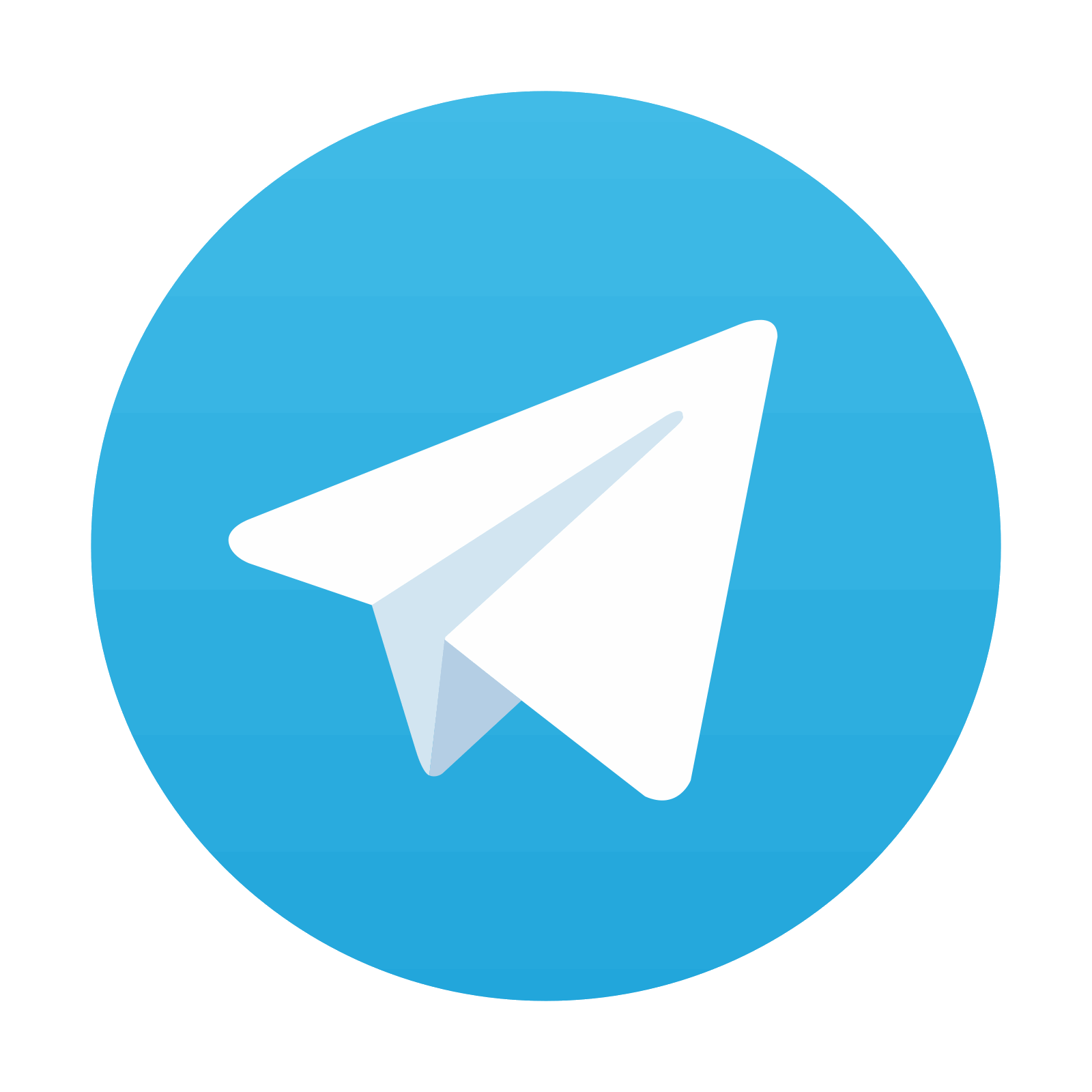
Fluorescence imaging with the QD light source. Reprinted from Ref. [12], copyright (2014), with permission from Elsevier. (a) Fluorescence excitation setup. (b) Schematic of the experimental setup. The emission from the light source QD (QD540) excites the sample QD (QD620). (c) (Left) Transmission spectra of the cyan filter and the red (magenta + yellow) filter, which were used as the excitation filter and the emission filter, respectively. (Middle) Emission spectra of the QD light source (QD540) before and after the excitation filter. (Right) Emission from QD620 before and after the emission filter. (d) Bright field (left), reference fluorescence (middle), and QD-excited fluorescence (right) images of SKBR3 cells (top) and MDA MB 435 cells (bottom)
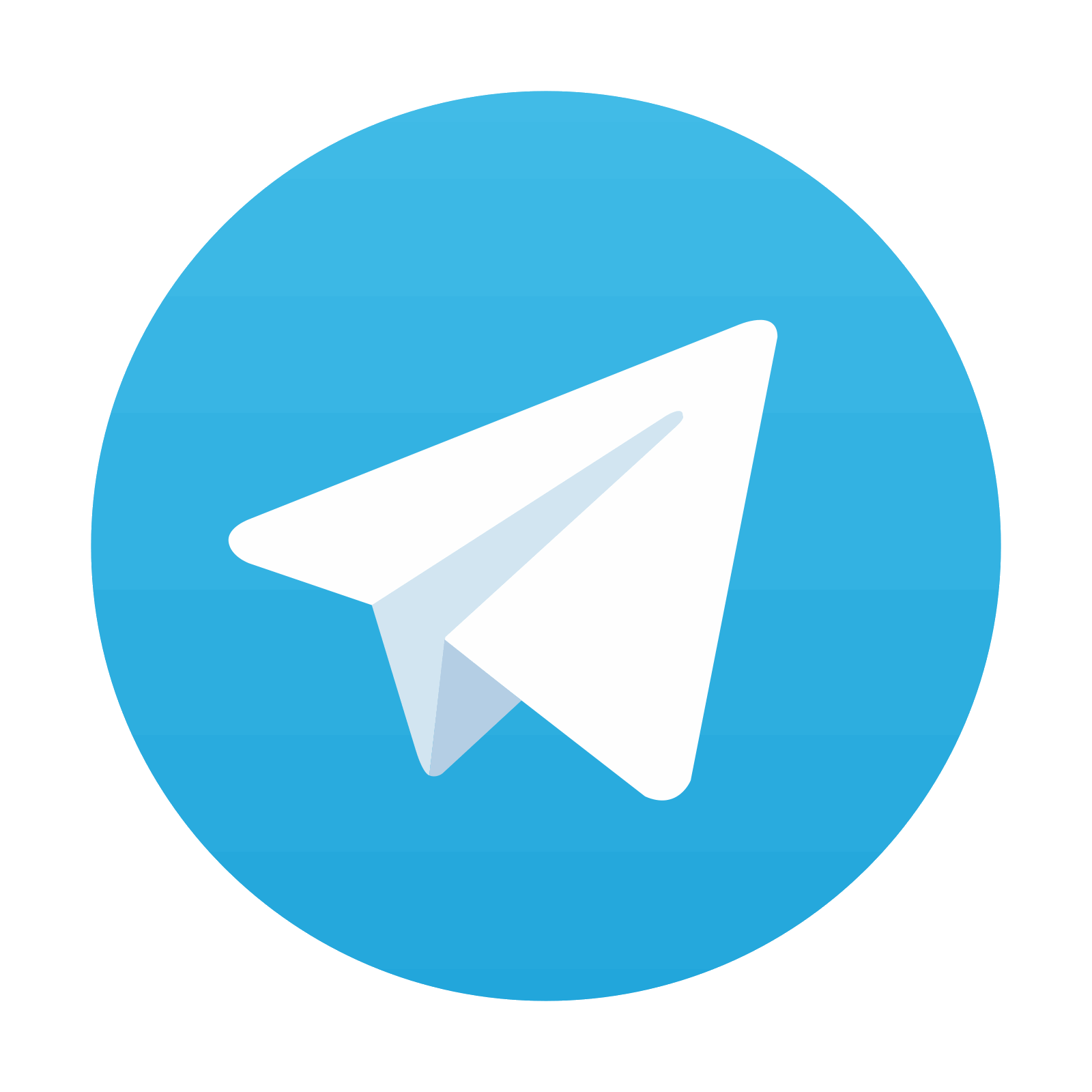
Stay updated, free articles. Join our Telegram channel
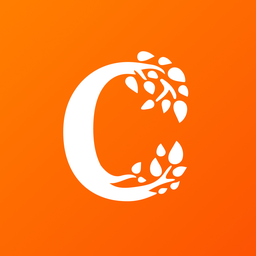
Full access? Get Clinical Tree
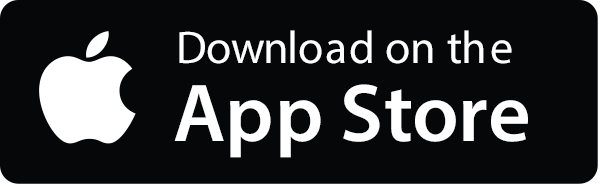
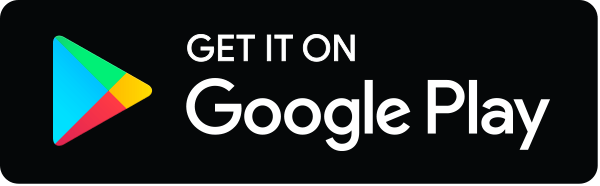
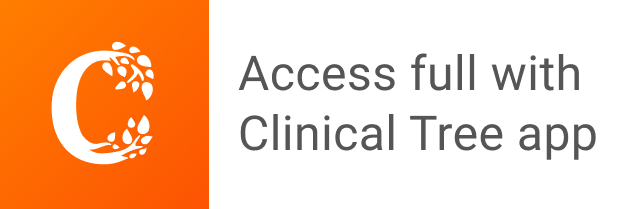