Fig. 1
Schematic representation of commonly used nanoparticle-based PET/MRI and SPECT/MRI probes
Table 1
Physical properties of nanoparticle-based PET/MRI probes
Nanoparticle | MRI component | PET component | Chelator | Core size (nm) | Hydrodynamic diameter (nm) | r 1 (mM–1s–1) | r 2 (mM–1s–1) | Application | Ref. |
---|---|---|---|---|---|---|---|---|---|
124I-Gd3N@C80 | Gd3+ | 124I | – | – | – | 70 | – | Tumor | [21] |
64Cu-NOTA-Au-IONP-Affibody | Iron oxide | 64Cu | NOTA | ~10 | 24.4 ± 2.0 | – | 143.2 (7T) | Tumor | [43] |
*As-SPION@PAA | Iron oxide | 71,72,74As | – | ~10 | 21 | – | 93.8 (4.7T) | Lymph node | [44] |
Magh-1-PNPs-NODA | Iron oxide | 68Ga | NODA | 8-12 | 44-55 | 0.5 | 182 (1.5T) | Pancreatic cancer | [45] |
MDIO-64Cu-DOTA | Iron oxide | 64Cu | DOTA | 7-8 | 62.7 | 16.8 | 83.9 (1.4T) | Atherosclerotic plaques | [38] |
SDIO-DO3A | Iron oxide | 64Cu | DO3A | 5.9 | ~60 | 14 | 72 (1.4T) | Atherosclerotic plaques | [46] |
Gd3+/Yb3+/Er3+ doped NaYF4 | Gd3+ | 18F | – | 22 × 19 | 28.2 | 0.40 (3T) | – | Nonspecific | [47] |
NOTA-OA-IONP | Iron oxide | 68Ga | NOTA | <15 | 66.3 ± 31.0 | – | 157 (4.7T) | Tumor | [48] |
cRGD-SPIO | Iron oxide | 64Cu | NOTA | ~10 | 68 | – | 101.9 (4.7T) | Tumor | [49] |
68Ga-SPIONs | Iron oxide | 68Ga | – | – | 30 | – | – | Sentinel lymph node | [50] |
Fe3O4@Al(OH)3 | Iron oxide | 18F | – | – | 21 | 4.9 | 121.9 (3T) | Nonspecific | [51] |
MnFe2O4@Al(OH)3 | Mn doped iron oxide | 18F | – | – | 21 | 8.2 | 20.1 (3T) | Nonspecific | [51] |
Co0.16Fe2.84O4@NaYF4 | Iron oxide | 18F | – | 10.3 | 44 | 5 | 102 (3T) | Sentinel lymph node (passive) | [13] |
89Zr-Feraheme | Iron oxide | 89Zr | – | 5-10 | 18.1 | – | – | Nonspecific | [39] |
69Ge-SPION@PEG | Iron oxide | 69Ge | – | 10 | 23 | – | 93.8 (4.7T) | Lymph node (passive) | [36] |
Gd3+/64Cu2+-DTPA-MSNs | Gd3+ | 64Cu | DTPA | 150 | 250 ± 76 | 17.6 (1.4T) | – | Nonspecific | [22] |
89Zr-ferumoxytol | Iron oxide | 89Zr | desferrioxamine | – | 17-35 | – | 89 (0.47T) | Lymph nodes | [52] |
PEMs | Iron oxide | 64Cu | DOTA | 100 (5 nm for single core) | 140 ± 7 | 2.15 | 265 (1.4T) | Tumor (passive) | [53] |
68GaNHFCNP | Iron oxide | 68Ga | NOTA | 15.3 | – | – | – | Target cancer cell though charge | [37] |
AGuIX | Gd3+ | 68Ga | NODA (68Ga), DOTA (Gd) | – | 2.5 ± 0.1 | 10.3 | 13.4 (1.4T) | Nonspecific | [20] |
Table 2
Physical properties of the reported nanoparticle-based SPECT/MRI probes
Nanoparticle | MRI component | SPECT component | Chelator | Core size (nm) | Hydrodynamic diameter (nm) | r 1 (mM–1s–1) | r 2 (mM–1s–1) | Application | Ref. |
---|---|---|---|---|---|---|---|---|---|
[59Fe]-SPIONs | Iron oxide | 59Fe | – | 5.0 ± 1.5 | 17 ± 6 | – | 97 ± 3 | Nonspecific | [34] |
111In-doped iron oxide | Iron oxide | 111In | – | 7.7 | 37.3 | – | – | Nonspecific | [33] |
99mTc-LBA-SPION | Iron oxide | 99mTc | DTPA | 12 | – | – | – | Human hepatoblastoma | [17] |
99mTc-NBRh1 | Iron oxide | 99mTc | – | – | 160.8 ± 1.3 | – | – | Tumor | [28] |
99mTc-USPION-RGD | Iron oxide | 99mTc | DTPA | 3.5 ± 1.2 | 10 | 8.2 | 20.1 | Tumor | [14] |
99mTc-AF-SPIONs | Iron oxide | 99mTc | – | 11 ± 2 | – | – | – | Lymph nodes (passive) | [27] |
99mTc-DTPA-USPIO-Annexin V | Iron oxide | 99mTc | DTPA | 3-5 | – | 8.2 | 20.1 | Vulnerable atherosclerotic plaques | [15] |
cRGD-conjugated IO-NPs | Iron oxide | 99mTc | – | 10 ± 2 | ~200 | – | – | Tumor | [12] |
177Lu-trastuzumab-iron oxide | Iron oxide | 177Lu | DOTA | 9.0 ± 0.5 | 41 ± 15 | – | – | Tumor | [35] |
99mTc-Au–Fe3O4 core-shell NPs | Iron oxide | 99mTc | – | 7.9 ± 0.4 | 11 | – | – | Nonspecific | [25] |
99mTc-Au–Fe3O4 dumbbell-like NPs | Iron oxide | 99mTc | – | 26.1 ± 1.6 | 35 | – | – | Nonspecific | [25] |
Radiolabled PEGylated SPIO | Iron oxide | 111In, 14C, 59Fe | DTPA | 15 | 40 ± 7 | – | – | Nonspecific | [54] |
Fe3O4–3H11- 125I | Iron oxide | 125I | – | – | 33.5 | – | – | Tumor | [16] |
99mTc-MNP | Iron oxide | 99mTc | – | – | – | – | – | Nonspecific | [55] |
125I-cRGD-USPIO | Iron oxide | 125I | – | – | 51.3 | – | – | Breast cancer | [30] |
131I-hVEGF siRNA/SilenceMag | Iron oxide | 131I | – | – | – | – | – | Hepatocellular carcinoma | [31] |
Fe3O4-Ag125I heterodimers | Iron oxide | 125I | – | 14 (Fe3O4) 9 (Ag125I) | – | 139.8 | Nonspecific | [41] | |
RGD-liposomes | Gd3+ | 111In | – | 182 | 2.7 | 16.2 (6.3 T) | Tumor | [19] | |
99mTc-DPA-ale-Endorem | Iron oxide | 99mTc | DPA | 5 | 106 ± 60 | – | 26 (9.4 T) | Nonspecific | [24] |
99mTc-SPIONs | Iron oxide | 99mTc | – | 11 | 18 | – | – | Sentinel lymph node (passive) | [23] |
111In-mAbMB-SPIONs | Iron oxide | 111In | DTPA | 15.6 | 76.6 | 0.59 | 468.57 | Tumor | [32] |
111In-liposomes | Gd3+ | 111In | – | – | 110.4–131.3 | – | – | Tumor | [18] |
99mTc-USPIO-bevacizumab | Iron oxide | 99mTc | DTPA | <20 | – | – | – | Hepatocellular carcinoma | [26] |
125I-fSiO4@SPIOs | Iron oxide | 125I | – | 6 | 20 | – | 165 (1.41 T) | Stem cell tracking | [29] |
2.1 Iron Oxide-Based Imaging Probes
Iron oxide nanoparticles (maghemite γ-Fe2O3 or magnetite Fe3O4) are commonly used platforms for constructing multimodal probes for PET/MR and SPECT/MR imaging because of their unique magnetic and biological properties. Iron oxide nanoparticles, in general, have an excellent biocompatibility profile at concentration ranges suitable for most diagnostic applications (0.1–10 μg/mL) because iron taken up by the liver and spleen is metabolized and joins the physiological iron pool. However, concerns for cytotoxicity could arise when concentrations of 100 μg/mL of iron oxide nanoparticles are exceeded [10] and adverse reactions such as hypotension and back pain have been reported for some formulations [11]. Besides biocompatibility, the large surface-to-volume ratio for iron oxide nanoparticles has been attractive for development of targeted agents. In addition, self-heating of these nanoparticles in the presence of alternating magnetic fields could facilitate controlled drug release or cytolysis of tumor cells [12], which has spurred interest in developing these magnetic nanoparticles as therapeutic agents for tumor treatments.
The synthetic designs of recently reported PET/MRI and SPECT/MRI nanoparticle-based multimodal probes are heavily dependent on the proposed usage of these probes in vivo. Some properties can be engineered into the core of the nanoparticle itself, and preparation of the iron oxide nanoparticles under controlled conditions (concentration of precursors, temperature, and solvent system) can modulate core properties (composition, hydrodynamic diameter, polydispersity, and shape). These properties of the core have been tailored to influence the biodistribution, blood circulation half-life, and magnetic anisotropy. For example, incorporation of cation dopants into the core of iron oxide nanoparticles can alter magnetic anisotropy and can provide additional imaging capability such as fluorescent imaging. Cui et al. developed hybrid inorganic composites and their variants: Fe3O4@NaYF4 core/shell nanoparticles, and bisphosphonate polyethylene glycol (BP-PEG)-coated Co0.16Fe2.84O4@NaYF4(Yb,Er)-BP-PEG and Fe3O4@NaYF4(Yb,Tm)-BP-PEG nanoparticles. These nanocomposites contained different lanthanide cation dopants Yb (sensitizer), Er, or Tm in NaYF4 shell for up-conversion fluorescence, and Co incorporated in the Fe3O4 core to adjust the magnetic properties of the nanoparticles [13]. Up-conversion fluorescence (emission of light at a shorter wavelength than the excitation wavelength) with emission peaks at 700 and 800 nm was observed for Fe3O4@NaYF4(Yb,Tm)-BP-PEG nanocomposites while emission signals centered at 525, 550, and 650 nm were obtained for Co0.16Fe2.84O4@NaYF4(Yb,Er)-BP-PEG nanoparticles upon excitation with a 980-nm laser. The high affinity of NaYF4 for [18F-fluoride] and the ability of bisphosphonate moiety to chelate 99mTc and 64Cu radiometals facilitated multimodal PET/MR/optical or SPECT/MR/optical imaging.
Surface modifications to introduce reactive moieties on the surface of the nanoparticles are often implemented. This includes attachment of chelators of MRI-active metals (e.g., Gd3+ and Mn2+) and radiometals for multimodal imaging; addition of ligands that allow targeting; conjugation with fluorescent dyes enabling optical imaging; and loading with drugs for delivery. The surface functionalization of reported SPECT/MRI and PET/MRI probes typically involves modification of the surface with abundant –NH2 or –COOH groups for conjugation using any number of conjugation chemistries. For example, the –NH2 groups in the common coating material poly(ethylene glycol) 2-aminoethyl ether acetic acid (NH2-PEG-COOH) can be activated by m-maleimidobenzoyl-N-hydroxysulfosuccinimidyl ester (sulfo-MBS) for subsequent functionalization with targeting groups through a thiol-maleimide linkage [14] (Scheme 1). A structural derivative of PEG, COOH-PEG-COOH, can be conjugated to amine-terminated ligands such as phospholipid-binding Annexin V [15], an anti-gastric cancer monoclonal antibody 3H11 [16], by activating the –COOH groups via NHS-based biolinkers such as 1-ethyl-3-(3-dimethylaminopropyl)carbodiimide hydrochloride (EDC)/sulfo-N-hydroxysuccinimide (sulfo-NHS). The –COOH of carboxymethyl dextran on the iron oxide nanoparticle surfaces can be conjugated to chelators or targeting ligands using EDC/NHS via carbodiimide coupling chemistry. Similarly, EDC/NHS chemistry can be used to conjugate asiologlycoprotein-targeted lactobionic acid to the free amine groups of dopamine [17].
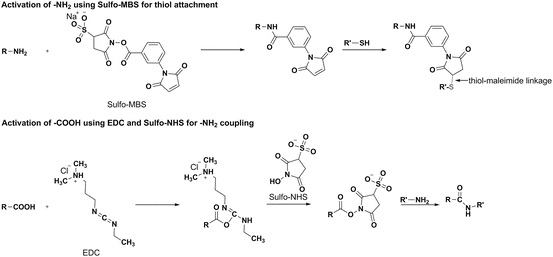
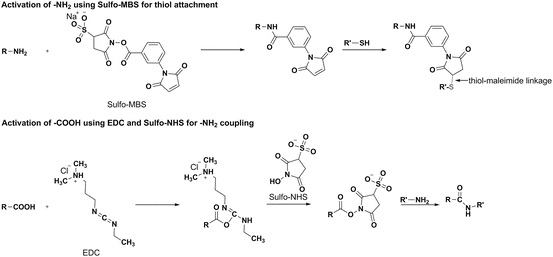
Scheme 1
Crosslinking strategies used in multimodal nanoparticle-based SPECT/MRI and PET/MRI probes for surface functionalization and subsequent ligand attachment
2.2 Non Iron Oxide-Based Imaging Probes
Liposomes have long been used as carriers for gadolinium-based MRI contrast agents. They are spherical vesicles composed of a bilayer of phospholipids with an aqueous interior [18]. By encapsulating large amounts of gadolinium ions, liposome-based nanoprobes have much higher longitudinal and transverse relaxivities compared with single molecule gadolinium chelates. The ability to accommodate different components (both lipophilic and hydrophilic compounds) makes the liposome an interesting vehicle for combined multimodal imaging and drug delivery systems. For example, de Vries et al. reported a multimodal liposome probe (182 nm in diameter) carrying gadolinium chelates, 111In chelates, rhodamine dye, and tumor-targeted cyclic RGD peptides (Fig. 2) [19]. The liposomes were prepared by the widely used thin film hydration method, where a thin lipid film is hydrated using buffer or other aqueous solutions to form the liposome. A biocompatible polymer coating, polyethylene glycol (PEG), was added to offer improved in vivo stability and blood half-life. In addition to SPECT/MRI dual modality imaging and SPECT-based quantification, the liposome cargo allowed in vitro and in vivo determination of local/cellular concentrations of gadolinium (given that the ratio of gadolinium to radionuclide of the probe was known), and permitted calculation of relaxivities in biological environments. A similar liposomal nanoprobe targeted to tumors using the RGD peptide and the neuropeptide substance P (binds to neurokinin-1 receptor), however, revealed only moderate tumor uptake and no additive effect of dual-targeting approach [18]. These findings suggest that the liposome-based probes have potential for further targeting optimization.