(1)
Department of Pathology, Faculty of Medicine, Kuwait University Health Science Center, Kuwait City, Kuwait
(2)
Department of Nuclear Medicine, Faculty of Medicine, Kuwait University, Kuwait City, Kuwait
5.1 Introduction
5.2.1 Blood Cells
5.2.2 The Bone Marrow
5.3 Erythropoiesis
5.3.1 Globin Chain Synthesis
5.3.2 Heme Synthesis
5.4 Iron Absorption
5.5 Ferrokinetics
5.5.1 Plasma Iron Clearance
5.5.2 Plasma Iron Turnover
5.5.5 Surface Counts for 59Fe
5.9.2 Vitamin B12 Radioassay
5.10 The Spleen
5.10.1 Spleen Imaging
5.12 Blood Platelets
5.1 Introduction
Nuclear hematology deals with the use of radionuclides or radiopharmaceutical agents in the study of the pathophysiology, diagnosis, and therapy of hematological diseases arising de novo in the hematopoietic tissues or as a consequence of some systemic diseases. This practice virtually began in 1940, when John Lawrence first used 32P to treat a young patient with chronic myeloid leukemia [1]. This was followed by the use of 32P as a radioactive label for red cells to measure blood volume [2]. From these modest beginnings, nuclear hematology has come a long way and evolved into a contemporary discipline as a very useful and often an essential investigative tool in many areas of hematology. Radionuclides are now widely used to label the formed elements of the blood (random labels) to trace their biological distribution, function, and life span in vivo as well as to study the proliferation and differentiation of hematopoietic progenitor and precursor cells in the bone marrow (cohort labels). The other major applications of nuclear hematology include the determination of spleen size, splenic sequestration of blood cells, and investigations relating to the absorption, metabolism, and utilization of hematopoietic nutrients such as iron, vitamin B12, and folate.
Many imaging techniques are being increasingly employed and explored in order to determine the anatomical distribution of hematopoietic tissues in the bone marrow and other organs and to evaluate their significance in the diagnosis and management of various hematological disorders. This chapter reviews the pathophysiological basis of the important applications of radiopharmaceuticals and radioisotopes in the practice of hematology.
5.2 Hematopoiesis and Hematopoietic Tissues
Hematopoiesis is a complex biological process which represents a unique paradigm of developmental biology and ontogeny in a replicating mesenchymal cell system – the hematopoietic system. The various blood cells develop from the stem cells by multiplication, differentiation, orderly maturation, and release of mature cells from the bone marrow to the peripheral circulation. A dynamic equilibrium is maintained between cell death in peripheral circulation and compensatory production of these cells in the bone marrow creating a steady state of “normal blood cell numbers” in physiological conditions.
The circulating blood cells, i.e., the red blood cells, leukocytes, and platelets, are highly specialized cells with distinctive morphology (structures) and functions. They are end-stage cells of their respective lineages and are destined to be lost from the circulation after a relatively brief time span of hours, days, or weeks. A steady state is attained, however, and the physiological range of their numbers is maintained by a continuous supply of newly formed cells (regeneration) from the blood-forming (hematopoietic) tissues.
5.2.1 Blood Cells
The red blood cells (mature erythrocytes), each approximately 8 μm in diameter, contain hemoglobin in a reduced (ferrous) state for successful gaseous exchange in the tissues. They circulate in the vascular system as flexible biconcave disks maintaining osmotic equilibrium against high intracellular hemoglobin concentration and differential concentration gradients of intra- and extracellular potassium and sodium. The energy required for this physicochemical stability is provided by ATP generated by the anaerobic glycolytic (Embden-Meyerhof) pathway and the hexose monophosphate shunt pathway generating reduced coenzymes NADH and NADPH, respectively.
The mature leucocytes (white blood cells) comprise two broad groups – the granulocytes and monocytes (phagocytes) and the lymphocytes (immunocytes). Normally, only the mature leucocytes are found in the circulating peripheral blood. These include mature granulocytes (neutrophilic polymorphonuclear leucocytes, eosinophils, and basophils) (Fig. 5.1a–c), monocytes (Fig. 5.2a, b), and lymphocytes (Fig. 5.3a, b). Both granulocytes and monocytes have phagocytic functions. With Romanovsky stain, polymorphonuclear neutrophils show faint but fine granules (neutral) (Fig. 5.1a), eosinophils show larger spherical red or pink granules (Fig. 5.1b), whereas basophils show many dark-staining granules (Fig. 5.1c) in their cytoplasm. The monocytes and all of these granulocytes have variable degrees of phagocytic functions.
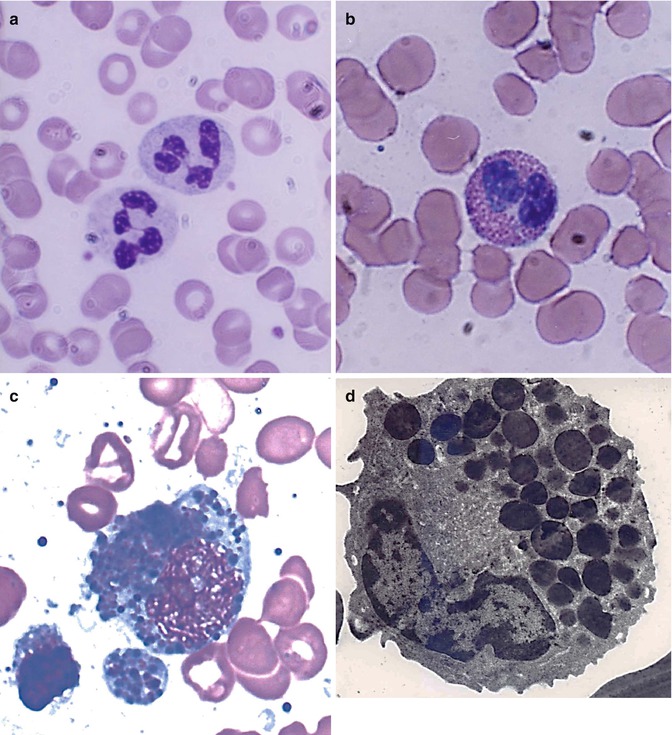
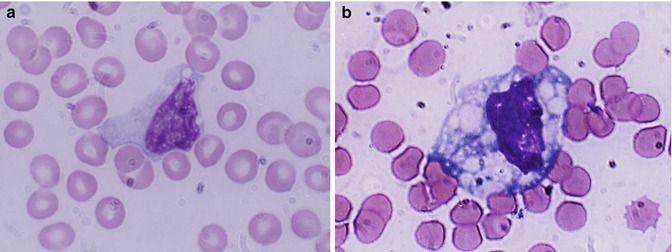
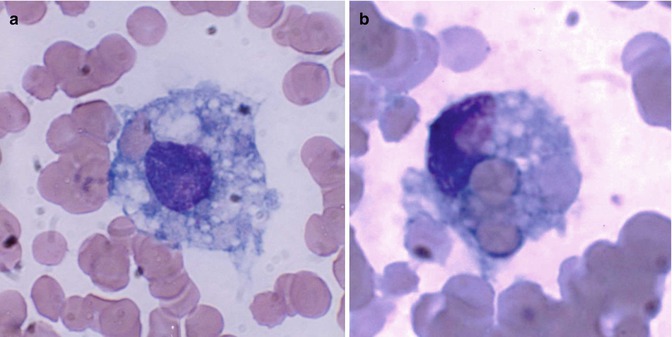
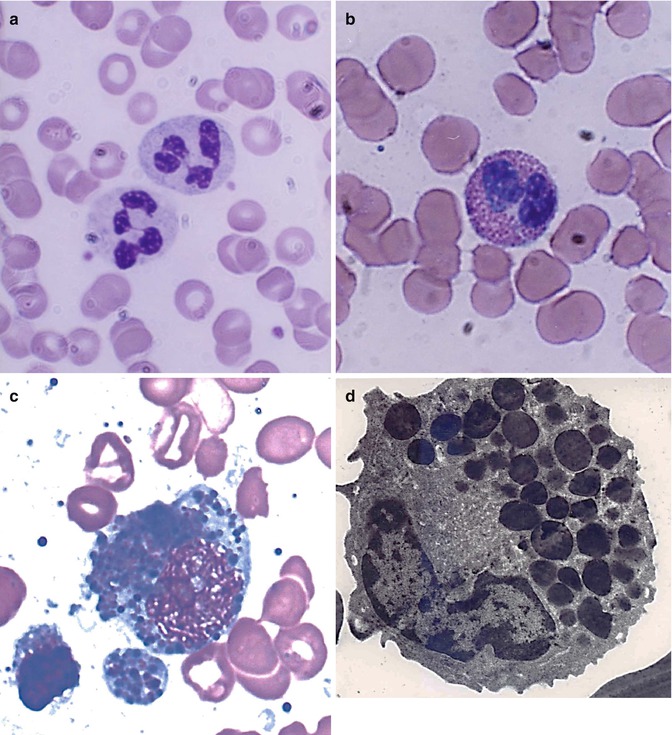
Fig. 5.1
(a) Shows neutrophilic polymorphonuclear leukocytes showing predominantly secondary or specific granules, which are fine and neither basophilic nor eosinophilic (neutral). In Romanovsky stain. (b) Shows an eosinophil, which is a polymorphonuclear leukocyte containing large eosinophilic prominent granules in the cytoplasm in Romanovsky stain. (c) Shows basophils, which are granulocytic leucocytes containing dark-colored (basophilic) granules in the cytoplasm in Romanovsky stain. (d) An electron microscopic picture of an eosinophil showing a large number of intracytoplasmic granules containing electron-dense crystalloid cores surrounded by lighter areas of matrix
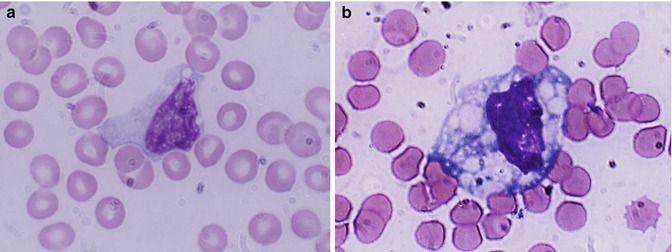
Fig. 5.2
(a, b) Shows a monocyte which are generally larger than neutrophils and have delicate fine nuclear chromatin, indistinct nucleoli, and thin membrane outline. The cytoplasm is abundant with irregular outline, light blue in color yielding a ground-glass appearance
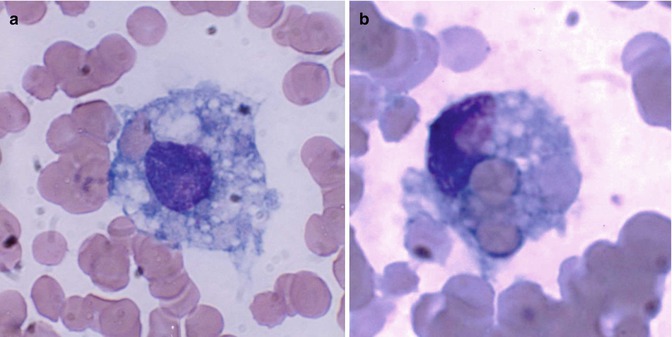
Fig. 5.3
(a, b) Shows a macrophages; these are large mononuclear cells (15–80 μm in diameter) with irregular cytoplasmic membrane outline suggesting amoeboid mobility, finely stippled nuclear chromatin, and spongy vesicular nucleus; (a) one macrophage shows a phagocytosed red cells; (b) a macrophage shows several phagocytosed red cells (erythrophagocytosis)
The mature neutrophils (Fig. 5.1a) contain several types of granules and other subcellular organelles. These include the primary or azurophilic granules and specific granules. The primary granules appear at the promyelocytic stage, gradually decrease as the cells mature, and contain many antimicrobial compounds which include myeloperoxidase, defensins, lysozyme, bactericidal permeability-increasing protein (BPI), and several serine proteases such as elastase, cathepsin G, proteinase 3, and others. The primary granules also contain organelles such as lysosomes, which fuse with phagocytic vesicles and deliver antimicrobial contents to the ingested organisms. Specific granules also called secondary granules of the neutrophils may fuse with phagocytic vesicles in inflammatory processes. The contents of these granules include lactoferrin, vitamin B12 binding proteins (transcobalamins I and III), plasminogen activator, collagenase, etc. These granules also play an important part in promoting chemotaxis and antimicrobial activities. The main functions of neutrophils consist in their mounting a protective response of the host to microbial infections. The neutrophils adhere to the endothelial cells (marginate) and then extend their cytoplasmic membrane (pseudopodia) into the endothelial cells lining the capillaries and thus emigrate into the tissues at the site of infection; the energy for this movement is generated by the activation of anaerobic glycolysis in the granulocytes. The plasma membrane of the involved neutrophils envelops the invading organisms or particles by its pseudopodia, which fuse around the organisms (phagocytosis) forming phagosomes. The phagosomes fuse with the contents of the azurophilic (primary) and specific (secondary) granules forming phagolysosomes facilitating microbicidal activities of the neutrophils. The phagocytosed microorganisms are killed and digested by synergistic oxidative (oxygen-dependent) and non-oxidative (oxygen-independent) reactions [3].
Eosinophils contain highly specialized and unique granules, each granule containing rectangular or square crystalloid core surrounded by lighter matrix in electron microscopic pictures (Fig. 5.1d). These granules contain a major basic protein (MBP) in the core, several other eosinophilic cationic proteins (ECP) in the matrix, and a number of proinflammatory cytokines. Eosinophils participate in allergic reactions, in defense against parasitic infections and removal of antigen-antibody complexes. These cationic proteins and proinflammatory cytokines have been implicated in tissue damage that occurs in asthma and other allergic conditions.
Basophils: Human basophils are round and have irregular short surface projections and many large dark-staining granules (Fig. 5.1c). Basophils are only occasionally seen in normal peripheral blood. The ultrastructure of these mature cells generally shows electron-dense cytoplasmic granules, prominent aggregates of cytoplasmic glycogen, and short, blunt irregularly distributed plasma membrane. There is no convincing evidence that mature basophils whether in the circulation or in the tissues retain mitotic capability or that basophils metamorphose into “mast cells” after entering the tissues. The dark cytoplasmic granules of basophils contain heparin, histamine, and minor quantities of other biogenic amines. These cells have receptors for IgE attachment.
Monocytes belong to the mononuclear phagocyte system which comprises monocytes, macrophages, and their precursors. These cells have a common origin and share similar basic morphology and functions. Monocytes are released from the bone marrow into the blood and after a variable yet short stay of 20–40 h migrate to different tissues either randomly or in response to chemotactic stimuli. In the tissues, these cells transform into macrophages after activation by microorganisms or other foreign particles. Monocytes are 10–12 μm in diameter, generally larger than mature neutrophils. They have large oval or indented nucleus with delicate nuclear chromatin, indistinct nucleoli, and thin membrane. When stained by Romanovsky stain, the cytoplasm appears abundant often with irregular outline and gray or light-blue color giving a ground-glass appearance (Fig. 5.2a, b) and may sometimes contain numerous vacuoles and rarely fine lilac-colored azure granules. The monocytes have a strong avidity for attachment to glass surfaces or polystyrene beads. Like polymorphonuclear neutrophils, monocytes are capable of amoeboid movement, chemotactic activity, and phagocytic and bactericidal functions.
Macrophages represent the tissue phase of the monocytes and are believed to arise by differentiation and transformation of emigrated blood monocytes in the tissues. Macrophages are large cells (15–80 μm in diameter) and irregular in shape with active amoeboid mobility of the cytoplasm which may contain many vacuoles. The nuclear chromatin is finely stippled and spongy and often appears vesicular. They are highly active cells with intensely powerful phagocytic and microbicidal activities. The phagocytic activity is often manifested by the presence of ingested red cells inside the phagocytes (Fig. 5.3a, b).
Lymphocytes tend to offer protection to the host by more subtle processes and are also known as immunocytes. These cells are formed from lymphoid stem cells in the primary lymphoid organs (i.e., thymus and bone marrow in postnatal life and yolk sac, liver, and spleen in prenatal life) independent of any antigenic stimulation but under the influence of several nonspecific cytokines. They are also formed in secondary or reactive lymphopoietic sites such as lymph nodes, spleen, lymphoid follicles of the alimentary and respiratory tracts, and other diffuse lymphoid tissues in response to antigenic challenges. The majority of the blood lymphocytes are small cells (8–10 μm in diameter) and have condensed nuclear chromatin occupying almost the whole of the cell. No nucleolus is usually visible (Fig. 5.4a). The cytoplasm is scanty and may appear as a bluish or moderately basophilic narrow rim in a small lymphocyte but more abundant in large lymphocytes. When stained by Romanovsky stain, the small lymphocytes show scant or no granules, but in large lymphocytes a small number of lilac-colored granules (azurophilic granules) may be seen; these granules are more numerous in the large granular lymphocytes (LGL). The latter cells are larger in size (10–12 μm in diameter), have pale blue more abundant cytoplasm containing peroxidase negative (azurophilic) granules, and constitute 10–12 % of the peripheral blood lymphocytes.
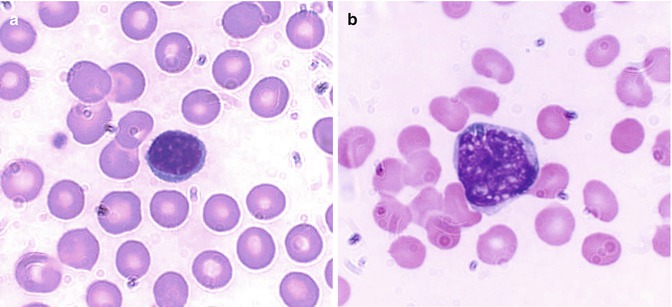
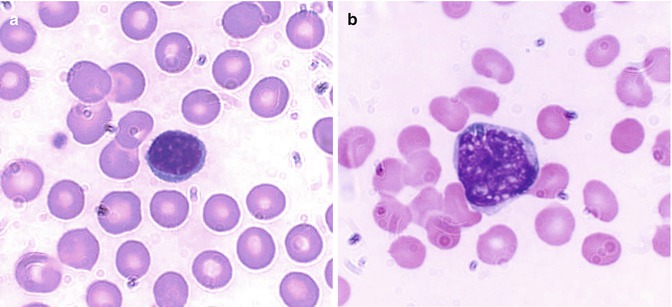
Fig. 5.4
(a) Shows a B-lymphocyte which is a relatively small round mononuclear cell (8–10 μm in diameter) and a nucleus occupying almost the whole of the cell usually with no nuclei, which has a bluish or moderately basophilic narrow rim of cytoplasm. (b) Shows a T-lymphocyte, which contains dense but uneven prominent chromatin structure with a shallow intranuclear indentation
The lymphocytes contain some mitochondria (visible under electron microscopy) for basic energy requirement of the resting cells (Fig. 5.5a). The lymphocytes in the peripheral blood are generally resting cells with mild or poor metabolic activities which are multiplied severalfold when these cells encounter an antigen, a mitogen, or an infection to which they respond by active transformation into a blastoid form – the “transformed lymphocytes” or “ activated lymphocytes.” These latter cells are as large as lymphoblasts and show intense pyroninophilia (strongly basophilic in Romanovsky stain), prominent nuclear chromatin, and one or more nucleoli (Fig. 5.6).
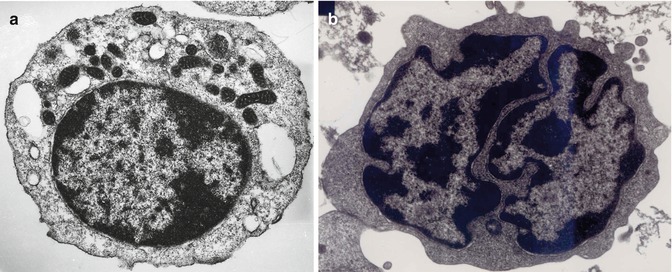
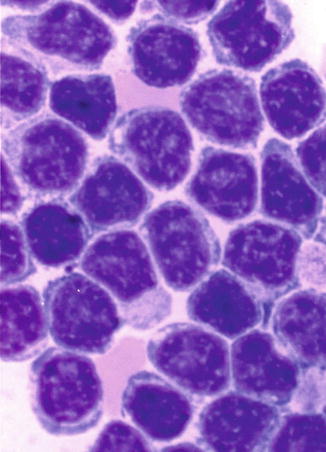
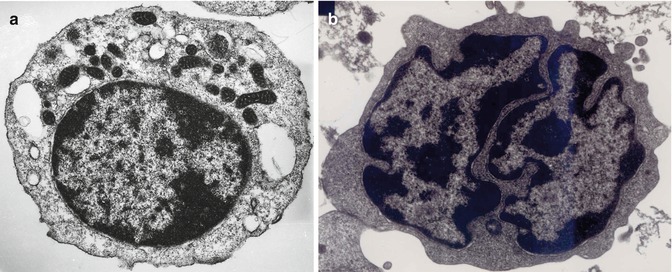
Fig. 5.5
(a) Transmission electron microscopic picture of a B-lymphocyte. The cell shows a small rim of cytoplasm, condensed nuclear chromatin, and several mitochondria in the cytoplasm. (b) Transmission electron microscopic picture of a T-lymphocyte, showing dense heterochromatin along the nuclear membrane and euchromatin in the remaining nuclear surface. The nucleus also shows deep indentations
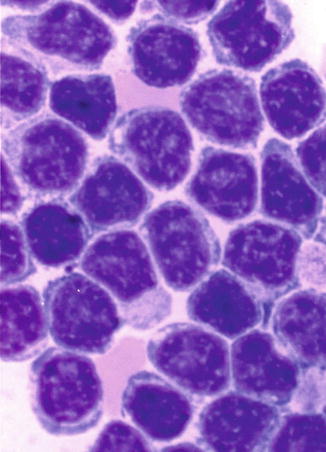
Fig. 5.6
This shows the morphological features of “transformed lymphocytes” that were generated by phytohemagglutinin stimulation of peripheral blood lymphocytes in short-term culture for 72 h. These lymphocytes turned larger, developed cytoplasmic basophilia, and revealed prominent nuclear chromatin and nucleoli
Lymphocytes (immunocytes) are functionally heterogeneous. The different subpopulations of immunologically competent lymphocytes have evolved in order to meet the requirements of diverse immune functions such as antigen recognition (self/nonself discrimination), clonal selection for antibody production, cell-mediated immune functions, immunological memory, and self-regulation. Broadly, B- and T-lymphocytes evolved to subserve these diverse immunological and biological activities. The various subpopulations of lymphocytes are identified and categorized by the presence of specific cell surface receptors (CD, cluster of differentiation antigens), which are detected by fluorescence-tagged specific monoclonal antibodies in a flow cytometer. B-lymphocytes are capable of synthesizing immunoglobulin (Ig) molecules which in the resting phase of the lymphocytes remain attached to the cell membrane and are used as identification markers. The B-lymphocytes are believed to arise from the bone marrow stem cells in the human, whereas in the avian species, these cells originate in the bursa of Fabricius; an equivalent organ in the human remains unidentified.
T-lymphocytes also originate in the bone marrow stem cells; the prothymocytic T-lymphocytes acquire CD7 antigen on their cell surface and then emigrate to the thymus, where epithelial and dendritic cells provide a microenvironment for their further induction and maturation. A mature T-lymphocyte often contains a dense but uneven prominent nuclear chromatin structure with one or more intranuclear indentation (Fig. 5.4b). The mature helper T-lymphocytes express CD4 and suppressor cells express CD8; other subpopulations of T-lymphocytes develop many other CD antigens. T-lymphocytes express cell surface receptors, αβ or γδ. Transmission electron microscopy of these cells reveals prominent basophilic cytoplasm, dense heterochromatin in the nucleus along the nuclear membrane, and euchromatin in the remaining area of the nucleus. There are also one or more deep nuclear indentations (Fig. 5.5b).
Plasma cells are a progeny of B-lymphocytes and actively synthesize immunoglobulins under appropriate antigenic stimuli during which they undergo morphological transformation. They can be morphologically distinguished from lymphocytes by their distinctive features. These cells are spherical or ellipsoidal in shape (5–25 μm in diameter) with diffusely basophilic deep blue cytoplasm but may sometimes have fine granular appearance. The nucleus is round or oval, often eccentrically placed, and has dense prominent, aggregated chromatin giving a “cartwheel” appearance. These cells have a well-defined perinuclear halo (clear zone) that contains the Golgi apparatus (Fig. 5.7a). The ultrastructure of plasma cells shows well-developed endoplasmic reticulum and perinuclear space with the Golgi apparatus (Fig. 5.7b).
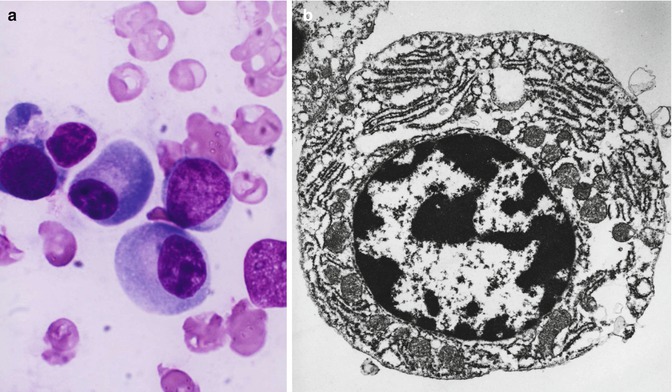
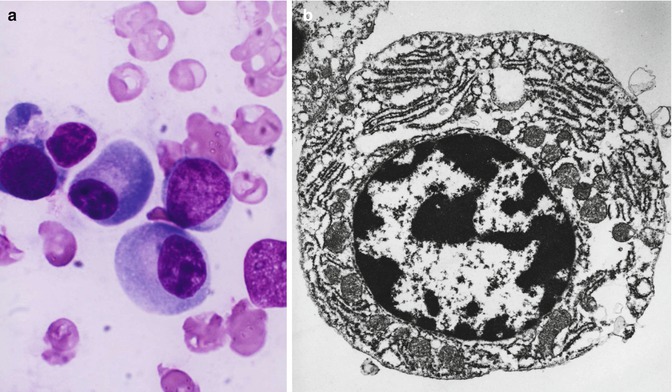
Fig. 5.7
(a) Shows the light microscopic morphology of a plasma cell in Romanovsky stain. The mature plasma cell shows strongly basophilic cytoplasm, eccentrically placed nucleus showing aggregated nuclear chromatin suggesting a “cartwheel” appearance. (b) Transmission electron microscopic picture of a plasma cell showing well-developed endoplasmic reticulum, eccentrically placed nucleus with condensed chromatin, and perinuclear zone with the Golgi apparatus
5.2.2 The Bone Marrow
The bone marrow is the principal hematopoietic tissue in the adult human being and contains the great majority of the hematopoietic stem cells and the hematopoietic inductive microenvironment that induces differentiation of the stem cells into each blood cell type, characteristic of the diverse cell lineages such as myeloid (granulocytic, monocytic, and erythroid cell) and lymphocytic series of cells. Considerable changes are known to occur in the site and nature of hematopoiesis at different stages of development from the embryo to the adult.
In the embryo, blood cell formation starts in the yolk sac mesoderm as primitive “blood islands” comprising a cluster of specialized mesodermal cells. These cells align themselves into peripheral endothelial cells and centrally located cells that produce a short-lived first line of primitive hematopoietic cells, the primitive erythroblasts at 2–3 weeks of prenatal life. By the 10th week of prenatal life, a second set of hematopoietic cells develop predominantly in the liver and the spleen from the stem cells that have presumably migrated from the yolk sac mesoderm and then constitute the definitive line of hematopoietic cells (predominantly erythropoietic cells). At this point, the fetal liver becomes the main site of blood formation, where erythropoiesis continues and granulopoiesis begins to appear (Fig. 5.8). The spleen is also an important organ for prenatal hematopoiesis, although it makes a much smaller contribution than the liver. Spleen and liver continue to produce blood cells until about 2 weeks before birth and make an important contribution to lymphopoiesis. From 20 weeks of fetal life on, the bone marrow becomes increasingly active in blood cell formation, and it constitutes the main hematopoietic organ at birth. During the first 2–3 postnatal years, the bone marrow shows very active hematopoiesis in all bones in the body. During childhood, there is a gradual replacement of active, hematopoietic (red) marrow by relatively inactive, fatty (yellow) marrow. This change starts in the diaphyses of long bones and extends toward the epiphyses (Fig. 5.9). In early adulthood, active hematopoietic marrow is confined to the epiphyses of the long bones and all areas of the flat bones (axial skeleton) such as the sternum, ribs, cranium, vertebrae, and pelvis. Even in these “active” hematopoietic tissues, fat cells constitute approximately half of the total marrow tissues (Fig. 5.10). The red and yellow marrow each constitutes half of the bone marrow weight. Since half of the red marrow is fatty, 75 % of the total bone marrow in the adult human is virtually adipose tissue [4]. The hematopoietic cells in the red marrow gradually recede with advancing age from about 60 % in the first decade to about 30 % in the eighth decade of life [5]. When there is an increased need for blood cell formation in response to a hematological stress (e.g., hemolytic anemias), there is an expansion of active hematopoietic tissues to the areas containing predominantly fatty, yellow marrow and sometimes to the liver and the spleen causing “extramedullary hematopoiesis.” The hematopoietic cells in the extramedullary hematopoietic foci develop from the stem cells which migrate to those sites from the bone marrow via the circulating blood [6].
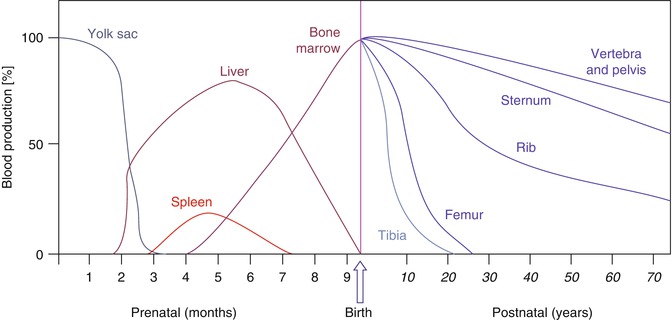
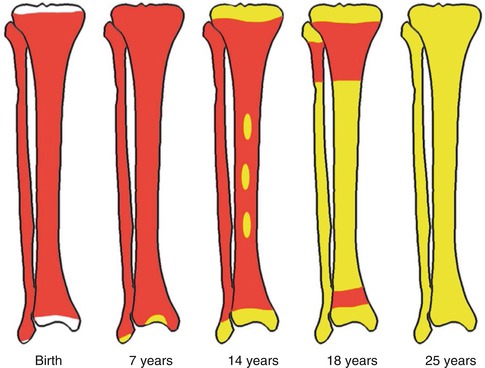
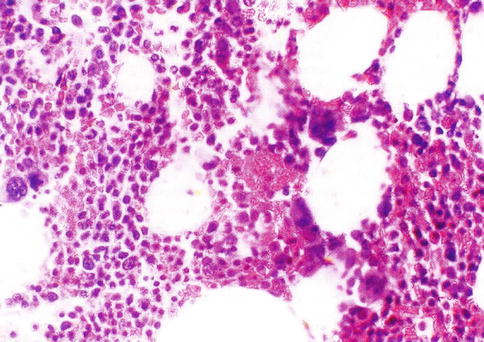
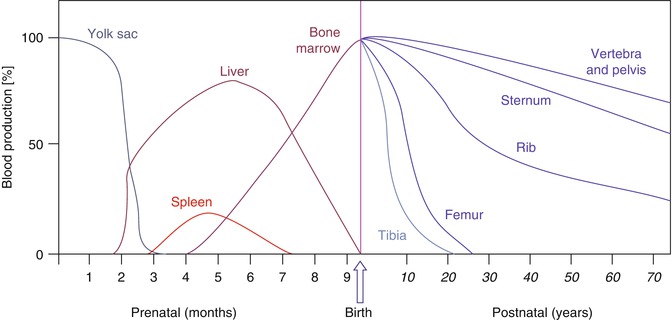
Fig. 5.8
The sites of pre- and postnatal hematopoiesis
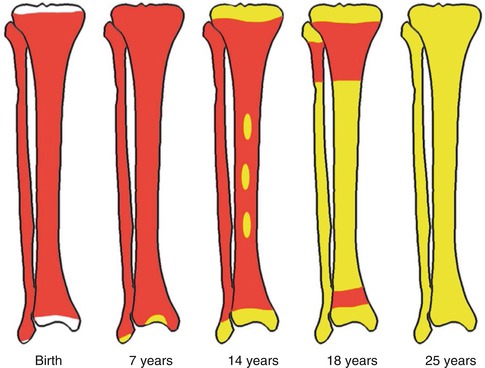
Fig. 5.9
Bone marrow distribution in long bones of the lower extremity illustrating changes during development over the years until the adult pattern is reached by about 25 years of age. (a) Birth, (b) 7 years old, (c) 14 years old, (d) 18 years old, and (e) 25 years old
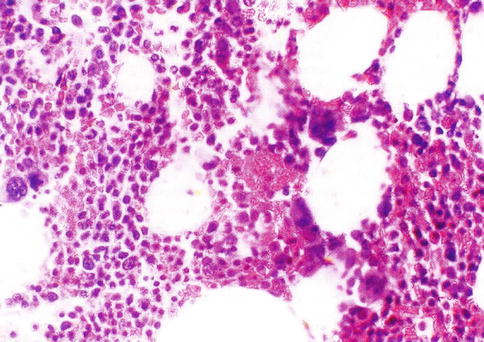
Fig. 5.10
Histological section of a bone marrow biopsy from an adult human being. At least 50 % of the marrow tissue shows fat cells and approximately 50 % hematopoietic cells. H & E
Bone marrow weighs approximately 3,000 g in normal adult men and 2,600 g in women. There is a dual blood supply to the bone marrow consisting of a periosteal capillary network and a nutrient artery that penetrates the bony shaft and divides into multiple branches in the marrow tissue. The blood flow through the bone marrow has been estimated to be about 10 ml/min/100 cm2 in normal volunteers, as assessed with positron emission tomography using a 15O-labeled CO2 steady-state technique. The blood flow was shown to be 2.5–3.5 times higher in patients with polycythemia vera (26.9 ± 4.6), chronic myeloid leukemia (25.2 ± 3.9), and myelofibrosis (35.1 ± 7.3) [6]. Blood flow was found to be in the normal range, however, in patients with aplastic anemia, chronic hemolysis, or chronic lymphatic leukemia which would suggest that there is no direct correlation between blood flow and bone marrow cellularity [7].
5.2.3 Hematopoietic Growth Factors
The development of hematopoietic cells depends on both (a) the genetically programmed innate cellular processes such as proliferation and maturation and (b) the induction and regulation of these processes by hematopoietic growth factors. A large number of these growth factors have been identified and chemically characterized and their amino acid sequences analyzed. The chromosomal locations of the responsible genes for most of them have been identified and cloned. While a comprehensive description of the cytokines is beyond the scope of this review, some general characteristics of these factors are relevant and useful to understand the functioning of the hematopoietic system. The hematopoietic growth factors (cytokines) are all glycoproteins, behave and function as hormones, and share a number of common properties.
T-lymphocytes, cells of the monocyte-macrophage system, endothelial cells, and fibroblasts are the major cellular sources of these growth factors in the body except for erythropoietin, which is produced predominantly in the kidneys. The biological functions of these growth factors are mediated through specific receptors on their target cells (hematopoietic cells and their precursors). They may act locally at the site of production or after they are absorbed into the circulating blood. One growth factor may act at one or more stages of hematopoietic cell maturation, and multiple factors may act on more than one stage of cell development. Several growth factors appear to exert synergistic action at multiple stages of development in the same lineage or even in more than one lineage but in a hierarchical manner (Fig. 5.11). The growth factors not only promote the proliferation, maturation, and functions of hematopoietic target cells but also inhibit the apoptosis of the target cells. The latter (i.e., apoptosis) represents a genetically programmed process of cell death that normally balances the rate of blood cell production and helps maintain a steady state. A large number of these cytokines are now available as recombinant DNA products for therapeutic and experimental applications.
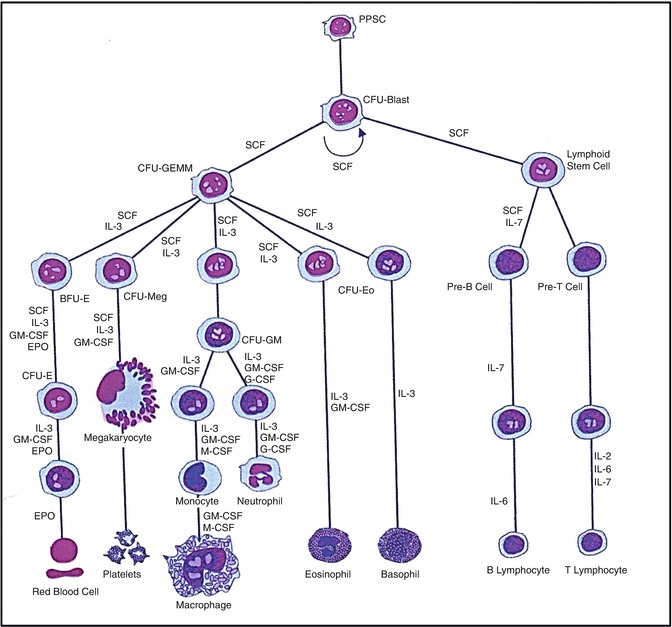
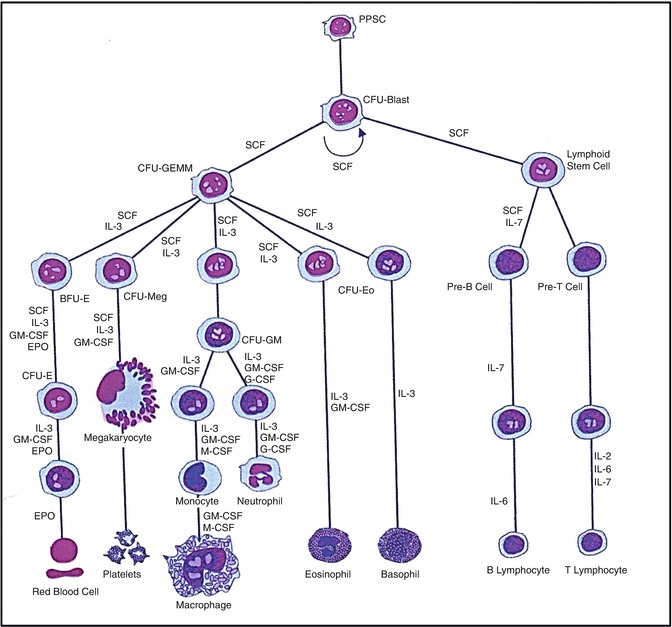
Fig. 5.11
A hematopoietic pluripotent stem cell (PPSC) and the different cell lineages that develop from it under the influence of various hematopoietic growth factors (cytokines) (Modified from [110] with permission)
5.2.4 Hematopoiesis and Hematopoietic Stem Cells
The factors or processes that induce the undifferentiated mesoderm to be committed to the development of multiple lineages of hematopoietic cells have only recently begun to be understood in some measure. The hematopoietic cells constitute a highly efficient system of hierarchical development of multilineage blood cells with specialized structural and functional characteristics (Fig. 5.11). The pluripotent stem cells conceptually develop from the undifferentiated mesodermal cells and are characterized by their capacity for proliferation, self-maintenance, or self-renewal. A replicate population of these stem cells gives rise to progenitor cells which become irreversibly committed to differentiation along one or the other hematopoietic cell lineages. These processes of cellular proliferation and differentiation appear to occur in a sequential manner and also possibly as overlapping events under the influence of a large number of hematopoietic growth factors. The flexibility inherent in these processes provides for enormous amplification of the cell systems on demand. The spleen colony-forming unit (CFU-S), a murine self-renewing transplantable stem cell described by Till and McCullough [8], is conceptually closest to the pluripotent stem cells. The successful development of in vitro colony-forming units in agar- or methylcellulose-based tissue culture media supplemented by biological fluids (which contain hematopoietic growth factors) has provided a very useful system for studying the stem cells. These technical developments led to the identification of several colony-forming units such as CFU-GEMM, CFU-GM, CFU-G, CFU-M, and CFU-E. These committed stem cells, in turn, generate the earliest morphologically recognizable cells in different hematopoietic cell lines (lineages) such as myeloblasts in the granulocytic, proerythroblasts in the erythroid cells (red blood cells), and promegakaryocytes in the platelet series. These progenitors, precursors, and other more mature cells are held in the hematopoietic microenvironment of the stroma by numerous adhesive molecules for which appropriate receptors are present in the hematopoietic cells and also in the stromal cells (Fig. 5.12). The microenvironment plays an important and perhaps essential function in sustaining hematopoiesis. It is believed that damage to the microenvironment of the bone marrow causes irreversible impairment of growth and proliferation of hematopoietic cells and may be responsible for bone marrow failure in a subset of patients with aplastic anemia and myelofibrosis.
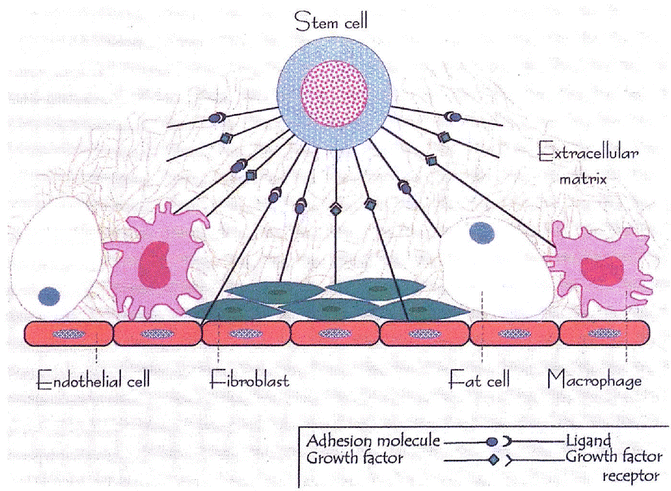
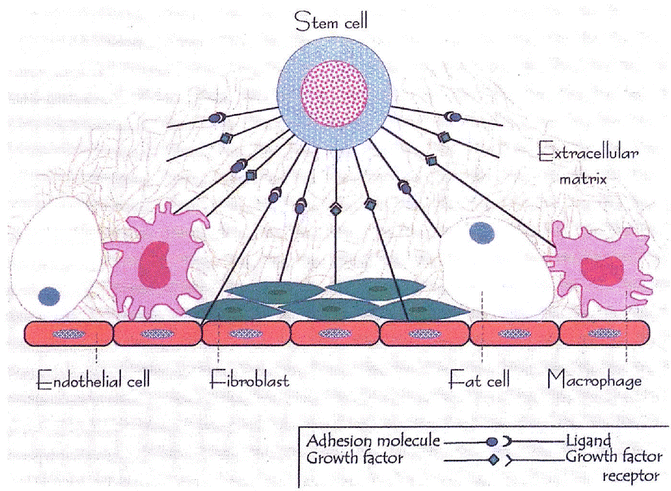
Fig. 5.12
The hematopoietic microenvironment provided by a stromal matrix on which stem cells and other hematopoietic progenitors and precursor cells divide and grow (Modified with permission from [110])
As the hematopoietic cells progressively grow and mature, the receptors for the adhesive protein molecules are downregulated, and the cells become less adherent and more mobile. Interestingly, the development of lineage specificity of the hematopoietic cells is associated with loss of some receptors and acquisition of others. Many CFU-S, though multipotent, do not appear to have long-term repopulating capability [9]. A cell or a group of cells that provide long-term hematopoietic reconstitution of radiation-ablated bone marrow including repopulation of all myeloid and lymphoid cell lineages are referred to as long-term hematopoietic repopulating units. These cells correspond to the totipotent or pluripotent stem cells conceptually or operationally. An in vitro assay system for hematopoietic stem cells can identify and quantify the cells in a test population that are capable of initiating long-term hematopoiesis in culture after seeding them onto irradiated stromal cell monolayers [10, 11]. These cells are referred to as long-term culture-initiating cells (LTC-IC) [10] and have been shown to grow and sustain production of multilineage progenitors of both myeloid and lymphoid series for many weeks in the presence of appropriate hematopoietic growth factors [10, 11]. Some recent reports indicate that human LTC-IC can be expanded substantially in vitro over a culture period of several weeks [12, 13].
5.2.5 Hematopoietic Cell Lineages
The bone marrow is normally the principal or the only site of blood cell formation in childhood and adult life in the human being. The developing hematopoietic cells grow in the stromal microenvironment outside the sinusoids in the bone marrow and when mature are released into the sinusoidal space (microcirculation of the bone marrow) and then into the general circulation. The hematopoietic cells comprise at least four lineages: (a) the granulocytic and monocytic, (b) the erythroid, (c) the megakaryocytic, and (d) the lymphoid cell lines. All of these cells apparently originate from a common stem cell (totipotent/pluripotent stem cell) which is believed to divide and differentiate initially into the multipotent stem cell, CFU-GEMM (common stem cell for granulocytic, erythroid, monocytic, and megakaryocytic series), and the lymphoid stem cell (Fig. 5.11). Further division and maturation of these progenitor cells follow, and these cells become irreversibly committed to differentiation along the morphologically and functionally distinctive lines of different blood cells under the influence of a number of hematopoietic growth factors and nutrients.
5.3 Erythropoiesis
The formation of mature red blood cells in the bone marrow occurs in different stages starting with the first stem cell progeny committed to erythroid differentiation and ending with the release of red cells into the circulation. The whole mass of erythroid tissue is conceived as a functional unit and is often referred to as the erythron comprising the mature red blood cells, the morphologically recognizable erythroid precursor cells, and their functionally defined (as erythroid colony-forming units) progenitors in the bone marrow. This functional concept of the erythron has contributed significantly to the understanding of the physiology and pathology of normal and abnormal erythropoiesis, respectively [9]. The earliest recognizable erythroid precursor cell in the bone marrow is the proerythroblast (pronormoblast in the normal bone marrow), which in the widely used Romanovsky stain appears as a large cell with dark-blue cytoplasm, a central nucleus, and prominent nuclei. This cell undergoes several divisions (usually four) and progressive maturation to give rise to the basophilic, the polychromatic, and the orthochromatic normoblasts, respectively. As these cells mature, they become smaller, the nuclear chromatin becomes more condensed, and the cytoplasm appears increasingly more hemoglobinized. The nucleus is finally extruded from the normoblast at the orthochromatic stage, and a reticulocyte is formed, which contains some ribosomal RNA and can still synthesize hemoglobin. The reticulocytes spend about 1–2 days in the bone marrow before they are released into the circulation, where they spend another 1–2 days mainly in the spleen to mature into red cells. A mature red cell is devoid of RNA and can no longer synthesize hemoglobin.
Normal human peripheral blood contains about 1–2 % reticulocytes (i.e., 25–75 × 109/l) and no normoblasts. Under physiological conditions, erythropoiesis is a well-balanced process in which the rate of red cell production is regulated so as to maintain a steady state and a relatively constant red cell mass. The glycoprotein hormone – erythropoietin which is a highly glycosylated polypeptide of 165 amino acids – is the major humoral regulator of erythropoiesis. Erythropoietin has been established as the major regulatory growth factor for erythropoiesis and is known to act on the committed erythroid stem cell – CFU-E – as well as on the other erythroid precursor cells, whereas the early stages of erythropoiesis up to the stage of BFU-E (burst-forming unit-erythroid) are independent of erythropoietin or are minimally influenced by this hormone. As cells in the CFU-E proliferate and differentiate into the red cell precursors giving rise to morphologically recognizable normoblasts of various stages (with Romanovsky stain), a number of biochemical events occur in these cells. These include increased synthesis of RNA, induction of globin gene transcription (mainly alpha and beta globin genes), increased uptake of calcium and glucose, synthesis of transferrin receptors, increased iron uptake, and synthesis of red cell membrane proteins [9, 14]. Hemoglobin synthesis continues throughout all stages of maturation of erythroblasts and also persists at a very low rate in the reticulocytes after the extrusion of the nucleus.
The process of differentiation of committed erythroid cell – the colony-forming unit-erythroid (CFU-E) – into the various stages of erythroid precursors (i.e., pronormoblasts and basophilic, polychromatic, and orthochromatic normoblasts, reticulocytes) is associated with activation of genes for hemoglobin synthesis. Thus, this process involves genes for at least three different biochemical pathways corresponding to the three essential components of the hemoglobin molecule – globin chains, protoporphyrin, and iron.
5.3.1 Globin Chain Synthesis
There are two distinct unlinked gene clusters for the two groups of globin polypeptide chains of hemoglobin in the human. The β-gene cluster (50 kb) (containing the linked genes ∈, Gγ, Aγ, δ, and β) is located on the long arm of chromosome 11, whereas the ζ, α2, α1, gene cluster is located on the short arm of chromosome 16. Like most mRNAs in the eukaryotic cells, the globin mRNAs are synthesized in a precursor form which is about three times as long as the finally processed template for protein synthesis. These precursor molecules undergo further processing in order to be converted to the final mRNA template including “capping” at the 5′ end of the molecule, polyadenylation at the 3′ end, and “splicing”; the latter process removes the intervening sequences or introns. The finally processed mRNA for globin chain synthesis contains 675–750 nucleotides [15]. The rate of globin chain synthesis has been shown to be regulated in a significant measure by heme [16, 17]. The presence of heme is known to stimulate globin chain synthesis in reticulocytes in vivo and in cell-free systems, and this is executed by a major effect exerted on the chain-initiation step in translation and to a smaller extent in transcription of globin mRNA or its processing [18]. In conditions associated with an absence or a deficiency of heme (e.g., iron deficiency), an inhibitor of globin chain synthesis accumulates in the system, which slows down or inhibits the rate of globin chain synthesis and functions as a rate-limiting factor [19–21].
5.3.2 Heme Synthesis
Heme is a ferrous complex of protoporphyrin IX, which is a tetrapyrrole ring compound and synthesized in the body in several sequential steps starting with the condensation of glycine and succinyl coenzyme A to yield δ-aminolevulinic acid (ALA) under the action of the enzyme ALA-synthase (ALAS) with pyridoxal-5-phosphate (vitamin B6) as a coenzyme. The enzyme ALAS is encoded by a gene located in chromosome 3; in erythroid cells, ALA synthesis is catalyzed predominantly by the erythroid-specific ALAS, which is coded by a gene located in the X chromosome. In the next step, two molecules of ALA undergo further condensation to form porphobilinogen, the primary building block for all natural tetrapyrroles. Four molecules of porphobilinogen condense through several steps of enzymatic reactions to form protoporphyrin IX in the mitochondrion, where protoporphyrin IX again combines with ferrous iron to yield ferrous protoporphyrin IX (i.e., heme). The latter reaction is catalyzed by the enzyme heme synthase (ferrochelatase) in the presence of the cofactor pyridoxal-5-phosphate (vitamin B6).
5.3.3 Essential Hematopoietic Nutrients
The hematopoietic cells constitute a cell renewal system, which produces a large number of cells daily to maintain the steady state of normal blood cell numbers and to meet the additional demands of increased cell production during stress. The biological processes of proliferation and maturation depend on a succession of carefully regulated biochemical events in the cells, which include turnover of DNA, RNA, and protein. These processes require a number of cofactors such as vitamin B12, folate, and vitamin B6 and also minerals such as iron, cobalt, copper, and manganese. While vitamin B12, folate, and vitamin B6 are required for the synthesis of DNA and RNA and for interconversions of amino acids, iron is an essential constituent of hemoglobin and is necessarily for erythropoiesis. Iron is also an important component of many enzyme systems including nucleotide diphosphate reductase, which converts the substrate nucleotide diphosphate to deoxynucleotide diphosphate in the pathway for thymine-DNA synthesis.
5.3.4 Iron Metabolism and Erythropoiesis
Iron metabolism is intimately related to hemoglobin synthesis and thus to erythropoiesis. Iron is the most abundant mineral micronutrient present in the human body. Although iron is one of the most common elements in the earth’s crust, yet iron deficiency anemia is the most common type of anemia all over the world. The total body iron content in normal adult men is approximately 50 mg/kg body wt., and in women, this is about 35 mg/kg body wt. Two-thirds of the total body iron exists as hemoglobin in the erythrocytes. Plasma iron is derived mainly from the reticuloendothelial (RE) cells and the macrophages which acquire iron by engulfing senescent and effete erythrocytes at the end of their life span (erythrophagocytosis) (Fig. 5.3a, b). The iron so obtained as a degradation product of hemoglobin by the RE cells generally becomes deposited as ferritin and hemosiderin (siderotic granules). Although monocytes lack transferrin receptors, these cells express these receptors, when they are transformed into macrophages. It is important to mention, however, that a small proportion of plasma iron comes from dietary sources after their absorption through the duodenum and jejunum. The plasma iron pool is the most important source of iron supply to the various body cells. Iron in the blood plasma is bound almost exclusively to the specific binding protein transferrin, a β−globulin which is synthesized in the liver and has a half-life of 8–10 days. Transferrin is the exclusive transport protein for iron delivering it to the cells of the erythroid series and to a smaller extent to other cells. Each molecule of transferrin can bind two atoms of iron and is normally one-third saturated with iron (i.e., transferrin saturation 33 %). Normal plasma contains 2.0–4.0 g transferrin per liter. In an average normal subject, the plasma iron concentration is approximately 18 μmol/l (100 μg/dl), and total iron binding capacity (TIBC) is approximately 56 μmol/l (300 μg/dl). The total transferrin-bound iron in the plasma amounts to approximately 4 mg; this cycles seven times each day to provide about 28 mg of transferrin-bound iron to be reutilized over and over again for hemoglobin synthesis and thus complete the internal iron cycle (Fig. 5.13). Transferrin delivers iron to the red cell precursors (proerythroblasts, early or basophilic normoblasts, intermediate or polychromatic normoblasts, late or orthochromatic normoblasts and reticulocytes) and to a smaller extent to other cells by binding to a specific cell surface receptor (transferrin receptor, CD71) (Fig. 5.14). Like many other receptors, the transferrin receptor is also a disulfide-linked transmembrane glycoprotein. This is encoded by a gene located in chromosome 3q26-qter [22]. Transferrin receptors can bind two types of transferrin molecules: diferric transferrin with a high affinity and monoferric transferrin with a somewhat lower affinity. As a result, diferric transferrin has a competitive advantage in delivering iron to the erythrocyte precursors [23]. During erythroid maturation, the number of transferrin receptors on the cell surface increases reaching a peak in the polychromatic or intermediate normoblasts. Only a few are found on BFU-E and a few more on CFU-E. The early or basophilic normoblasts are reported to have about 300,000 receptors on each cell increasing to about 800,000 at the polychromatic or intermediate stage. This number decreases further to about 100,000 on the reticulocytes, but with considerable variability depending on the degree of maturation of an individual reticulocyte. The rate of iron uptake by the erythroid cells is related to the number of transferrin receptors on their surface. The mature erythrocytes have very few or no transferrin receptor on their surface and, therefore, cannot take up iron from the plasma. The erythroid precursor cells appear to shed their receptors gradually as they mature possibly by proteolytic cleavage [24]. The shed (free) transferrin receptors appear in the plasma in a concentration that correlates with the rate of erythropoiesis [25, 26]. The transferrin receptors in the plasma can be quantitated with considerable accuracy. An increase in the plasma concentration of transferrin receptors is considered to be a sensitive indicator of iron deficiency.
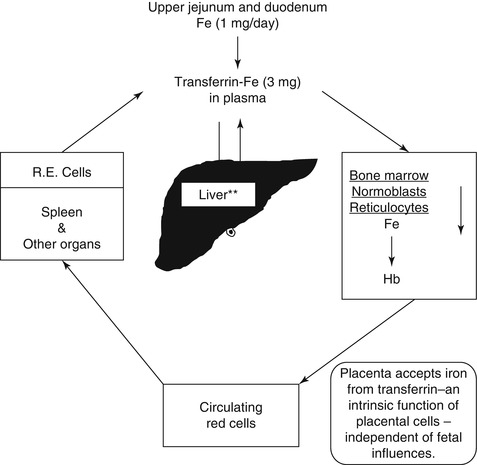
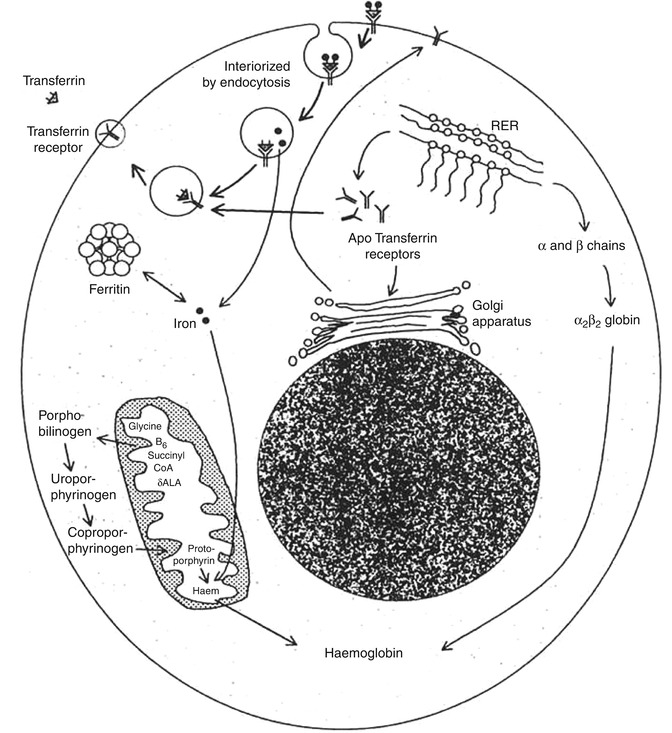
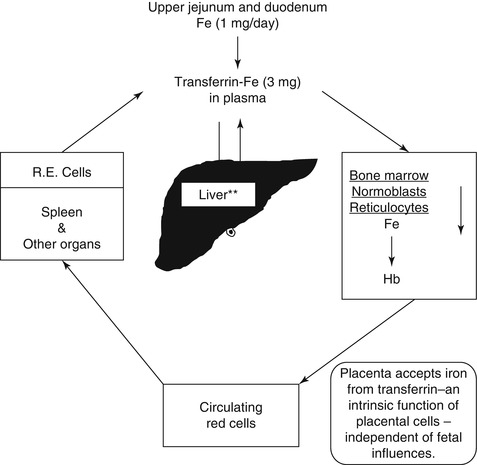
Fig. 5.13
The internal metabolic cycle of iron in the human being
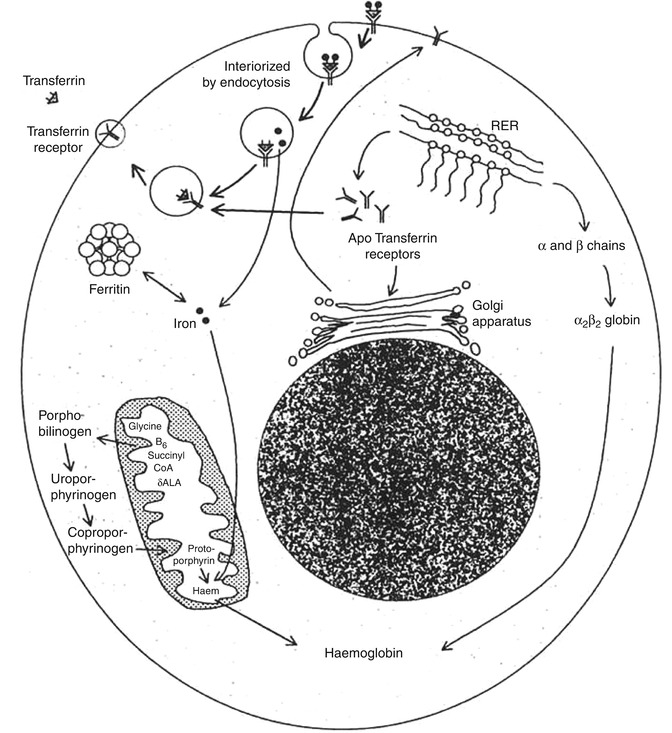
Fig. 5.14
The transferrin-iron cycle with postulated mechanism of iron uptake by the cells that have transferrin receptors (CD-71) on their cell surface (Das KC, unpublished work)
The mechanism of transport of iron from the surface of the erythroid precursor cells to their interior is interesting and subtle. The transferrin molecules with iron bound to them form a complex with the transferrin receptors on the cell surface and are then interiorized by endocytosis into vesicles. The contents of the vesicles are then acidified, and the iron is released from the complex leaving the iron-free transferrin still bound to the receptor. The iron then enters the cytosol, and the vesicle containing the transferrin-receptor complex is transported back to the surface of the cell, where the neutral pH causes the release of the apotransferrin to the plasma. Normally, 80–90 % of the iron that enters the cell is taken up by the mitochondria and is incorporated into heme. Most of the remaining iron becomes bound to ferritin and remains in storage as siderotic granules. The overall rate of entry of iron into the erythroid precursor cells is intimately related to the rate of heme synthesis [27].
5.3.5 Intracellular Regulation of Iron
Intracellular iron metabolism appears to be regulated by a complex mechanism which allows the cells to acquire iron as they need and to avoid the toxic effects of excess iron. They do so by regulating the synthesis of transferrin receptors and apoferritin. Cells containing adequate iron synthesize apoferritin and inhibit the synthesis of transferrin receptors, thereby limiting the cellular entry of iron; on the other hand, iron-deficient cells accelerate the synthesis of transferrin receptors and thus promote the increase of cellular entry of iron but inhibit the synthesis of apoferritin, minimizing its diversion to the storage pool. This is achieved at the transcriptional level. The mRNAs for transferrin receptors and for apoferritin contain stem-loop structures that constitute iron-responsive elements (IREs); five such IREs are present in the mRNA for transferrin receptors and one in the mRNA for apoferritin. The IREs bind the two iron-regulatory proteins (IRPs), IRP1 and IRP2, respectively. This binding produces opposite effects on the two mRNAs. While the stability of mRNA for transferrin receptor increases with consequent increased synthesis of transferrin receptors, the translation of apoferritin is inhibited.
5.3.6 Qualitative and Quantitative Aspects of Erythropoiesis
As stated earlier in this chapter, erythropoiesis entails a number of sequential events such as (a) proliferation and maturation of erythroid cells in the bone marrow, (b) hemoglobin synthesis in these cells including in the reticulocytes, and (c) release of mature erythrocytes into the circulation. However, not all erythroid precursor cells develop into mature red cells to be released into the peripheral blood even in normal individuals. It has been shown that about 5–10 % of the total erythroid precursor cells in the normal bone marrow die prematurely during maturation and are engulfed by the macrophages giving rise to ineffective erythropoiesis. These processes of premature intramedullary cell death and ineffective erythropoiesis are significantly increased in a number of hematological diseases:
1.
Megaloblastic anemia due to deficiency of vitamin B12 and/or folate or caused by their metabolic inhibitors
2.
Myelodysplastic syndrome
3.
Congenital dyserythropoietic anemias
4.
Myelofibrosis
5.
Thalassemia major
6.
Hypoplastic/aplastic anemia
7.
Bone marrow infiltration by metastatic neoplasms
8.
Leukemias, lymphomas, and myeloma
9.
Therapy with cytotoxic, antineoplastic drugs
10.
Anemias associated with chronic diseases
They constitute the major pathogenetic mechanism by which anemia and other cytopenias are caused. The apparent increase of cell mass of the erythroid precursor cells in the bone marrow yielding a decreased myeloid/erythroid ratio (often referred to as increased total erythropoiesis) contrasts with the decreased output of mature red cells into the circulation in most of these disorders with a few exceptions: For example, hypoplastic and aplastic anemias are characterized by a marked decrease of bone marrow cells involving all cell lines including erythroid precursor cells with a consequent decreased output of all mature blood cells including red blood cells (i.e., ineffective erythropoiesis). Although the etiology and mechanism (s) of bone marrow failure in these disorders may vary, the final outcome is similar and is manifested as ineffective hematopoiesis (and erythropoiesis) with the development of pancytopenia in the peripheral blood.
There are several biochemical markers of ineffective erythropoiesis which often provide useful indications for diagnosis. These include elevated serum level of unconjugated bilirubin (i.e., breakdown products of hemoglobin) and high serum level of lactate dehydrogenase (LDH) with a preponderance of the anodic fraction (LDH1), which is released as a result of increased intramedullary destruction of red cell precursor cells. The morphological examination of the bone marrow shows hyperplasia of erythroid precursor cells (erythroid hyperplasia) with a low reticulocyte count in the peripheral blood indicating ineffective erythropoiesis. These bone marrow changes explain the ferrokinetic findings of rapid plasma iron clearance with poor iron incorporation into the red blood cells. However, in hypoplastic and aplastic anemias, plasma iron clearance is slower than normal, but the fraction of injected radioactive iron incorporated into the newly formed red cells is reduced because of the reduced quantum of red cells produced in the bone marrow; this disorder is, therefore, associated with both anatomical and functional reduction and failure of erythropoiesis.
The metabolic cycle of iron is intimately related to hemoglobin synthesis and erythropoiesis. The use of radionuclides in studying iron metabolism helps in tracing the movement of iron in the metabolic cycle and can monitor the transport of iron, its uptake by hematopoietic tissue in the bone marrow and other organs, and the site, quantum, and nature of erythropoiesis. Radioactive iron (59Fe) is used to study the following aspects of iron metabolism and the kinetics of erythropoiesis (ferrokinetics):
1.
The gastrointestinal absorption of iron
2.
The distribution of 59Fe radioactivity following intravenous injection of a standard dose of radioactive iron
3.
The imaging of radioactive iron uptake by the bone marrow and other organs
5.4 Iron Absorption
The assessment of absorption of orally administered iron by the gastrointestinal tract constitutes theoretically a very important component of the study of iron status and iron metabolism in a patient. A small quantity of radioactive iron (59Fe) usually in the form of ferric chloride, mixed and diluted with nonradioactive iron (FeSO4, 7H2O) and a reducing agent (such as ascorbic acid) in a known volume of aqueous solution, is administered by mouth to the patient after an overnight fast; the patient is not allowed to take anything by mouth for further 3 h after the oral test dose of radioactive iron. The subsequent part of the procedure is cumbersome and involves collection of feces passed by the patient during the following 7 days or so and measuring the radioactivity of the radioiron excreted in the stool in a gamma scintillation counter against a control solution of 1 ml of the radioactive iron solution which is given to the patient to drink at the onset of the test. The absorption of iron is calculated as the difference between the intake of radioactive iron and its excretion in stool collected for a week after the test and is expressed as the percentage of ingested radioiron retained.
As stated earlier in this chapter, ingested iron is absorbed by the mucosa of the duodenum and jejunum by mechanisms that are complex and only partially understood. The rate of absorption is affected by a host of factors including the nature of the diet; the presence of chelators, reducing agents, and other interacting factors; intestinal mobility and function; and the state of erythropoiesis. Because of these dietary and biological variables, the interpretation of absorption data based on a small test dose of soluble inorganic iron is difficult in the context of deficiency or disorders of iron metabolism. The average iron absorption in normal subjects is reported to vary between 10 and 30 % of the ingested test dose; in iron-deficient states, the absorption is markedly increased depending on the severity of deficiency and perhaps the degree of transferrin saturation. The validity of the results of iron absorption tests also depends on the reliability of complete collection of stool samples until <1 % of the ingested radioactivity is excreted in 1 day’s collection of stool samples. For these reasons, the oral absorption test for iron is not considered to be of much clinical convenience and value, and it is sparingly used in clinical conditions.
5.5 Ferrokinetics
Iron turnover studies with intravenously injected radioiron (59Fe) provide the best obtainable indications of the movement of iron in the metabolic cycle, of the degree of total erythropoietic activity, and of the intensity of effective and ineffective erythropoiesis. However, injected iron is also taken up to some extent by the RE cells in the liver, spleen, and bone marrow, where it may be deposited as storage iron in the form of ferritin and hemosiderin, and also by the circulating reticulocytes. The ferrokinetic data yield several types of semiquantitative and quantitative information:
1.
Plasma iron clearance (i.e., the clearance of injected radioiron from the plasma)
3.
Iron utilization by newly formed red blood cells (RCU)
4.
Surface counting to measure the uptake and turnover of iron by various structures and organs (i.e., sacrum, liver, and spleen)
5.
Erythrocyte (red blood cell) iron turnover (EIT)
In these studies, approximately 5–10 ml of plasma is obtained aseptically from freshly collected heparinized blood of the patient. Radioactive iron (59Fe) in the form of ferric chloride is added to the plasma which is then incubated at room temperature for about 15 min. The radioiron becomes bound to the plasma transferrin, which is then injected intravenously into the patient. A 2-ml sample of blood is collected from the patient at 5 min, and another four to five samples are collected into heparin or EDTA at intervals of 15 min for 1–2 h after the injection of radioiron and then one sample daily for 10–15 days thereafter with heparin or EDTA as anticoagulant. The 59Fe radioactivity in the plasma and red cells of these samples is measured, and the plasma iron concentration and volume of packed red cells (VPRC) are determined.
5.5.1 Plasma Iron Clearance
The radioactivities of 59Fe in the plasma samples collected at different intervals are plotted on the Y-axis and the time intervals (minutes) on the X-axis on log-linear graph paper. The radioactivity at the time of radioiron injection is derived by extrapolation of the initial slope to zero. The time taken for the plasma radioactivity to decrease to half (50 %) of its initial level (T½) plasma clearance is derived from this graph (Fig. 5.15). The plasma iron clearance rate is related largely to the mass of erythroid cell population in the bone marrow and, therefore, apparently to the total erythropoietic activity and to some extent to the activity of the RE cell system in the liver, spleen, and bone marrow. It has been estimated that approximately 90 % of all body transferrin receptors are normally in the erythron and the remaining 10 % are mainly in the liver. In normal individuals in the steady state, 59Fe plasma clearance (59Fe T½) varies considerably. Our own studies of 25 clinically and hematologically normal subjects have shown that plasma 59Fe T½ ranges from 55 to 125 min (Table 5.1). A rapid plasma 59Fe clearance (i.e., shorter plasma 59Fe T½) would indicate increased total erythropoietic activity usually associated with an increase of erythroid precursor cell mass as seen in patients with megaloblastic anemia, myelodysplastic syndrome, thalassemia major, and iron-deficiency anemia. On the other hand, a slow or delayed plasma iron clearance (i.e., longer plasma 59Fe T½) occurs in patients with aplastic and hyperplastic anemias, which are characterized by reduced erythropoietic activity and depletion of erythroid precursor cell mass in the bone marrow with or without reduction of other cell lineages. In hematological neoplasias including leukemias and in myelofibrosis and myelosclerosis, the plasma iron clearance rate is variable depending on the degree of erythropoietic activity in the bone marrow and the presence or absence of extramedullary erythropoiesis; however, in these conditions, the plasma iron clearance rate is more often high (i.e., plasma 59Fe T½ is shorter; i.e., clearance is rapid) than low or normal (Das KC, unpublished data, 1990).
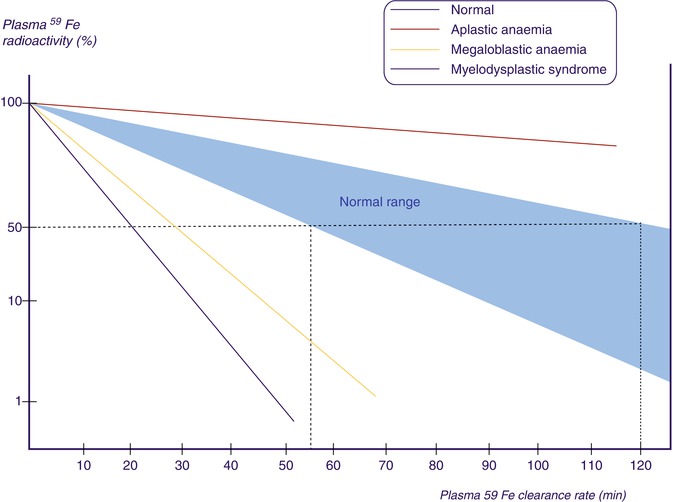
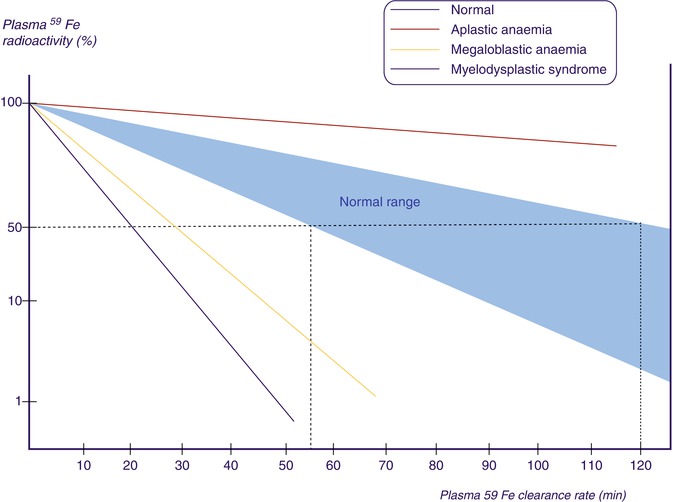
Fig. 5.15
Plasma iron clearance. 59Fe radioactivity in plasma at 5, 10, 20, 30, and 60 min after intravenous injection of a dose of radioiron (59Fe). The T½ (i.e., time in which 59Fe activity declined to 50 % of the zero time radioactivity)
Table 5.1
Ferrokinetic patterns in various types of hematological disorders
Plasma 59Fe clearance T½ (min)a | PIT (mg/day/dl) | Maximum RCU (%) | EIT (mg/day/dl) | |
---|---|---|---|---|
Normal | 55–125 (75) | 0.65–0.76 (0.71) | 80–90 (85) | 0.51–0.66 (0.58) |
Aplastic anemia | 180–360 (250) | 0.60–0.75 (0.60) | 14–20 (14) | 0.09–0.25 (0.12) |
Megaloblastic anemia | 30–45 (35) | 4.8–7.5 (6.3) | 15–30 (22) | 0.95–2.0 (1.5) |
Myelodysplastic syndrome | 35–50 (40) | 5.0–6.9 (6.2) | 10–35 (25) | 0.68–1.4 (1.1) |
Myelofibrosis | 30–47 (36) | 2.5–6.2 (4.8) | 14–20 (18) | 0.78–1.5 (1.3) |
5.5.2 Plasma Iron Turnover
The parameter of plasma iron turnover (PIT) is also referred to as the plasma iron transport rate [28, 29]. As mentioned above, the plasma iron clearance does not take into account the concentration of plasma iron and at the most yields a semiquantitative concept of the rate of movement of plasma iron to the erythropoietic tissue in the bone marrow and to the RE cell system. In determining the PIT rate, the plasma iron concentration is related with the plasma iron clearance rate to obtain quantitative data on the rate at which iron leaves the plasma per unit time and unit volume of blood. This is expressed as a total daily rate (i.e., mg or μmol of iron/l/day).
The computation of PIT is done according to the following formula:




PIT is a frequently used parameter in the ferrokinetic profile of patients with disorders of iron metabolism and anemias. It is considered to be a reasonably good indicator of total erythropoiesis and generally correlates with total nucleated red cell mass. Its clinical usefulness is limited, however, by several physiological constraints: (a) In calculating PIT, the blood volume is assumed to be normal, which can be amended by measuring the blood volume separately and expressing the results incorporating the blood volume in the calculation. (b) PIT does not take into consideration the fact that some iron leaves the plasma pool to enter the extravascular space (i.e., extravascular flux, EVF). (c) As stated earlier in this chapter, the plasma iron pool has both monoferric and diferric transferrins; diferric transferrin has a greater affinity for transferrin receptors and delivers twice as much iron per molecule of transferrin to the cells. For these reasons, the PIT rate tends to increase as the serum iron level increases or as the transferrin saturation increases [28, 29]. PIT is generally found to be increased in iron-deficiency anemia, hemolytic anemias, megaloblastic anemia [30], thalassemia major, and myelosclerosis. In aplastic anemia, the PIT is either normal or decreased, but it may also be increased when the plasma iron concentration is very high. The measurement of PIT has limited clinical usefulness for the reasons stated above and because of the fact that this parameter does not distinguish between effective and ineffective erythropoieses.
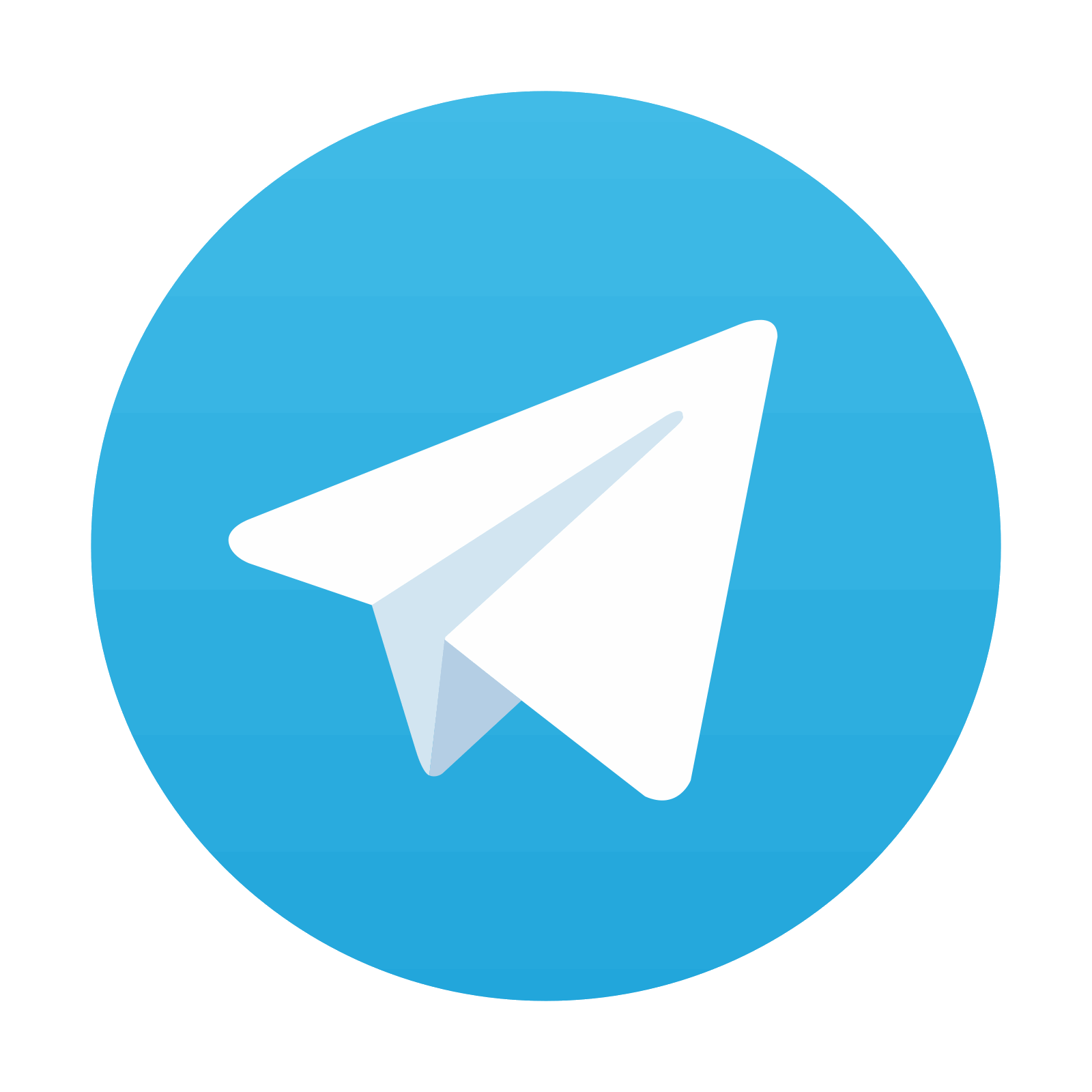
Stay updated, free articles. Join our Telegram channel
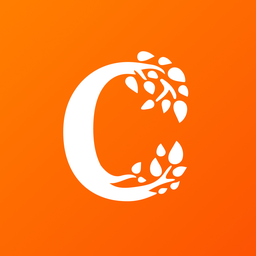
Full access? Get Clinical Tree
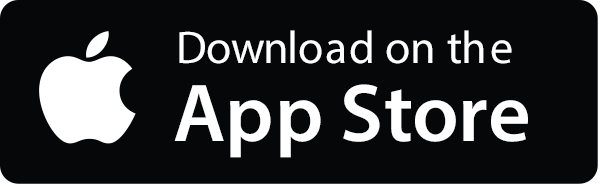
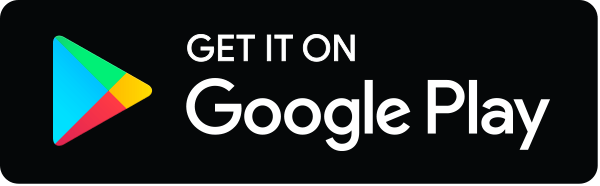