Cancer group
ACCIS (%)
SEER (%)
Total
72
75
Leukemias
73
74
Lymphomas and reticuloendothelial neoplasms
84
83
CNS and miscellaneous intracranial and intraspinal neoplasms
64
66
Sympathetic nervous system tumors
59
66
Retinoblastoma
93
95
Renal tumors
84
90
Hepatic tumors
57
56
Malignant bone tumors
61
68
Soft-tissue sarcomas
65
73
Germ cell, trophoblastic, and other gonadal neoplasms
84
87
Carcinomas and other malignant epithelial neoplasms
89
89
Others and unspecified malignant neoplasms
74
–
The most common tumors among the under 15-year-olds are leukemias, at 34%, brain tumors, at 23%, and lymphomas, at 12%, according to the International Classification of Childhood Cancer [2]. The most frequent single diagnoses are acute lymphoblastic leukemia, astrocytoma, neuroblastoma, non-Hodgkin’s lymphoma, and nephroblastoma. There is considerable variation between countries. Incidence rates range from 130 (British Isles) to 160 cases (Scandinavian countries) per million children. Incidence rates have shown an increase over time since the middle of the last century. In Europe, the yearly increase averages 1.1% for the 1978–1997 period and ranges from 0.6% for the leukemias to 1.8% for soft-tissue sarcomas [2].
Nuclear medicine is important in the diagnosis, staging, and long-term surveillance of a number of pediatric cancers. Skeletal scintigraphy is used to evaluate primary skeletal cancers, such as osteosarcoma and Ewing sarcoma, and nonskeletal cancers, such as neuroblastoma, lymphoma, medulloblastoma, rhabdomyosarcoma, and retinoblastoma. Metaiodobenzylguanidine scintigraphy is valuable in examinations of children with neuroblastoma, and [18F]FDG-PET/CT is useful in lymphoma, thymoma, and embryonal cell tumors.
[18F]FDG-PET imaging especially has an emerging role in the assessment of children with cancer. The conventional staging evaluation of these children includes magnetic resonance imaging (MRI) or CT of the primary tumor and regional nodal beds, chest CT for detection of pulmonary metastases, and technetium-99m methyldiphosphonate (99mTc-MDP) bone scan for detection of bone metastases. The Response Evaluation Criteria in Solid Tumors (RECIST), introduced in 2000 as an alternative to the World Health Organization (WHO) criteria, provide a standard, reproducible, and objective method of assessing the efficacy of solid tumor therapies [3]. Unfortunately, they rely on changes in unidimensional tumor measurements to define tumor response or progression. Solid malignancies, such as bone sarcomas and cystic soft-tissue sarcomas, may respond well to chemotherapy without substantially changing in size. Furthermore, sarcomas do not shrink or grow in a uniform manner; therefore, unidimensional measurements may not accurately reflect response or progression. Tumors that respond poorly to therapy may be followed for months before a unidimensional measurement increases significantly. Meanwhile, patients are exposed to toxic but ineffective chemotherapy and are likely to have a diminished probability of survival. [18F]FDG-PET, on the other hand, provides a three-dimensional assessment of viable tumor tissue based on metabolic activity. A decrease in tumor glucose uptake after treatment suggests a reduction in the number of viable tumor cells or a decrease in metabolism of the tumor tissue. Therefore, [18F]FDG-PET may offer a more sensitive evaluation of the response or progression of some pediatric solid malignancies than anatomic imaging modalities [4].
Pediatric Brain Tumors
Epidemiology
Intracranial tumors are the most common solid neoplasms in children and the second most common malignancy of childhood after leukemia. Among children younger than 19 years, the prevalence rate for all pediatric brain tumors is 9.5 per 10,000, while the incidence rate is 29.1 cases per 1,000,000 [5].
Pediatric brain tumors differ from adult tumors on various grounds. About 60–70% of all pediatric brain tumors, including astrocytomas, medulloblastomas, and ependymomas, develop in the infratentorial compartment. The remaining 30–40% of tumors are supratentorial and consist of gliomas, optic pathway tumors, hypothalamic tumors, and craniopharyngiomas. The reason why pediatric brain tumors have a propensity to occur in the posterior fossa has not yet been elucidated.
In contrast to those that occur in adults, most gliomas that occur in children are low-grade gliomas. Moreover, in the pediatric age, mixed (glioneuronal) and atypical (xanthoastrocytoma) tumors are frequent as well as lesions with very low or questionable evolving potential (hamartoma). Young age is a favorable prognostic factor for malignant gliomas. In adults, the majority of high-grade gliomas are primary and show amplification of the epidermal growth factor receptor (EGFR) gene, which encodes a tyrosine kinase involved in cell replication. In contrast, in pediatric patients, high-grade gliomas more commonly derive from low-grade gliomas and often have mutations in TP53 (a gene encoding for p53 protein) but seldom display amplification of EGFR [6].
Many types of supratentorial pediatric brain tumors are well delineated; they have a moderate tendency to infiltrate neighboring tissue and are usually accessible to total resection. However, some pediatric brain tumors may not be accessible surgically because they are close to vital brain structures. This typically occurs in medulloblastoma, a primitive neuroectodermal tumor that originates from the cerebellum, close to the brainstem and to the fourth ventricle.
Finally, 5–10% of brain tumors in childhood are thought to have a genetic predisposition, while the proportion is much lower in adults. Hereditary brain tumors occur in some familial cancer syndromes, such as the Li-Fraumeni syndrome. In adults, hereditary brain tumors are associated with rare hereditary syndromes, such as tuberous sclerosis, neurofibromatosis types 1 and 2, and familial adenomatous polyposis.
Symptoms
The diagnosis of brain tumor may be challenging in children because tumor signs and symptoms may resemble those of more common inflammatory or infectious diseases. Recurrent bouts of headache, nausea, or vomiting without focal deficits are frequently observed in children. For medulloblastoma, early symptoms are secondary to increased intracranial pressure due to blockage of the fourth ventricle. The child typically becomes lethargic, with repeated episodes of vomiting and morning headache. In later stages, the child develops stumbling gait, frequently falls, complains of diplopia, and develops papilledema and sixth cranial nerve palsy.
[18F]FDG Scan
The preparation and acquisition procedures are very similar to those for adult studies. Systemic sedation may be necessary for very young children, to eliminate motion during data acquisition. Dynamic scans are rarely performed.
An important consideration in pediatric PET scanning is limiting the radiation burden. The procedure guidelines of the Society of Nuclear Medicine recommend an administered activity of [18F]FDG ranging from 5 to 10 MBq per kg of body weight, from a minimum dose of 14 MBq for a 3D acquisition in a 3-kg infant to a maximum of 363 MBq for a 2D acquisition in a 68-kg patient [7]. Good hydration and frequent urine voiding are recommended to reduce bladder toxicity. The absorbed dose to the brain in infants is about 0.24 ± 0.05 mGy/MBq, a higher value than in adult subjects, because of the higher glucose consumption in the infant brain than in the adult brain [7].
[18F]FDG PET Studies
In 1992 [8], Hoffman et al. reported the [18F]FDG-PET results of 17 pediatric patients with posterior fossa brain tumors. [18F]FDG uptake was correlated to tumor histology. Increased [18F]FDG uptake was associated with more malignant and aggressive tumor types. Heterogeneity of [18F]FDG uptake was associated with previous therapy, including radiation therapy and chemotherapy. The authors concluded that [18F]FDG-PET would likely be an important adjunct in the management of pediatric posterior fossa tumors and predicted a rapid dissemination of PET technology for pediatric applications [8].
Nevertheless, PET is not routinely used for the clinical management of pediatric brain tumors. Literature remains relatively scarce on this matter, and the difference between adult and pediatric studies cannot be merely attributed to differences in prevalence of the disease [9].
Borgwardt et al. studied 38 untreated pediatric patients with primary CNS tumors using PET with [18F]FDG and, when possible (n = 16), with [15O]water to measure tumor perfusion. Image processing included coregistration to MR in all patients. The [18F]FDG uptake in tumors was semiquantitatively calculated with a region-of-interest approach. They found a positive correlation between [18F]FDG uptake and malignancy grading. On the contrary, there was no correlation between [15O]water uptake and histological grade, a finding that was attributed to uncoupling between glucose metabolism and blood flow. Digital PET/MR coregistration combined to image fusion improved the information on the tumor location in 90% of cases. PET altered management in 77% of patients with brain tumor [10].
Pirotte et al. reported their experience at the Erasme Hospital pediatric center where they examined with PET and [18F]FDG about 400 pediatric patients with brain tumor between 1995 and 2005. In their retrospective analysis, they included 126 patients in whom pre- and postoperative MR imaging showed limitations for assessing the evolving nature of an incidental lesion, selecting targets for biopsy and delineating tumor tissue for surgical resection. In this group of patients (about one-third of the whole case series), PET was expected to be most useful. They found that PET was helpful to (1) make a more appropriate decision in incidental lesions by detecting tumor/evolving tissue; (2) better differentiate indolent and active components of the lesion; (3) improve target selection and diagnostic yield of stereotactic biopsies, especially when performed in areas such as the brainstem or the pineal region; (4) provide prognostic information; (5) better delineate ill-defined tumor borders; (6) increase the number of tumor resections and the amount of tumor tissue surgically removed; (7) improve detection of tumor residues in the operative cavity at the early postoperative stage; (8) facilitate the decision for early second-look surgery for optimizing the radical resection; and (9) improve the accuracy of the radiosurgical dosimetry planning [11].
Utriainen et al. used PET with [18F]FDG and [11C]methionine to examine whether metabolic characteristics could be used as an index of clinical aggressiveness. SUV values for both tracers were compared with histopathology and selected histochemical features. The accumulation of both [18F]FDG and [11C]methionine was significantly higher in high-grade than in low-grade tumors, but a considerable overlap was found. High-grade tumors showed higher proliferative activity and vascularity than the low-grade tumors. In univariate analysis, [18F]FDG SUV, [11C]methionine SUV, and apoptotic index were independent predictors of event-free survival [12].
Childhood Lymphoma
Lymphoma accounts for 10–15% of all childhood malignancies and is the third most common cause of cancer, after leukemia and brain tumors. Non-Hodgkin’s lymphoma (NHL) is more frequent than Hodgkin’s lymphoma (HL) (about 60% and 40%, respectively) [13].
Hodgkin’s Lymphoma
HL, exceptional before the age of 2 years (median age: 10–12 years), usually presents with asymptomatic enlarged lymph node(s). The malignant cell in HL is the Reed-Sternberg cell, located in the tumor masses in a background of inflammatory cells and fibrosis. Four histologic subtypes of HL are described: lymphocytic predominance, mixed cellularity, lymphocytic depletion, and nodular sclerosis. Nodular sclerosis is the most common subtype, affecting 60% of children, whereas the lymphocytic depletion subtype is very rare. During therapy, residual masses are frequent and usually correspond to fibrosis and/or necrosis [14], especially in the nodular sclerosis subtype of HL because of its abundant fibrotic component [15]. Extent of disease at staging must be evaluated as accurately as possible because treatment is related not only to stage but also to each site of disease in order to plan conformal radiotherapy directed to all involved sites.
According to the Cotswold revision of the Ann Arbor classification, stage I is defined as involvement of a single lymph node region. Stage II has involvement of two or more lymph node regions on the same side of the diaphragm. Stage III has involvement of lymph node regions on both sides of the diaphragm. Stage IV has extranodal involvement, such as bone, liver, or lung disease. Involvement of the spleen is considered to be lymph node involvement. Each stage is also classified by the presence or absence of symptoms. “A” indicates that the patient is asymptomatic; “B” indicates that the patient has at least one of the following systemic symptoms: inexplicable weight loss of more than 10% within the last 6 months, unexplained persisting or recurrent temperature above 38°C, and/or drenching night sweats. “E” stage is defined as involvement of a single extranodal site contiguous or proximal to known nodal sites. Clinical and imaging evaluation must be performed at the end of therapy to confirm that therapy has been fully effective and has achieved complete response.
Major therapeutic progress has been made in HL, with current cure rates ranging from 90% to 95% for all stages [16, 17]. Most treatment regimens for childhood HL consist of chemotherapy and radiotherapy, with current efforts to reduce the radiation dose from 40 to 20 Gy as well as reducing the size of the field of radiation therapy and the cumulative doses of cytotoxic agents [18]. One of the main objectives of treatment is now to minimize late toxicity without compromising the cure rate.
Non-Hodgkin’s Lymphoma
NHL in childhood is significantly different from NHL in adults, as, in almost every case, it corresponds to high-grade malignancies with frequent invasion of the bone marrow and central nervous system [19]. In children, the predominant subtypes are Burkitt’s lymphoma, large B-cell lymphoma, and primary mediastinal B-cell lymphomas, followed by lymphoblastic lymphoma and anaplastic large-cell lymphoma [20]. NHL is very rare before the age of 2 years and has a peak incidence at the age of 7 years. NHL requires urgent treatment, consisting of combination chemotherapy (without radiotherapy) and neuromeningeal prophylaxis of recurrences by intrathecal chemotherapy. Most cases are extensive at diagnosis corresponding to stages 3 or 4 of the St. Jude Children’s Research Hospital classification (Murphy staging) [21].
Stage I disease is defined as a single tumor or nodal area outside of the abdomen and mediastinum. Stage II is a single tumor with regional lymph node involvement, two or more tumors or nodal areas on one side of the diaphragm, or a primary gastrointestinal tract tumor (completely resected) with or without regional node involvement. Stage III consists of tumors or lymph node areas on both sides of the diaphragm, or any primary intrathoracic (mediastinal, pleural, or thymic disease) or extensive intra-abdominal disease, or any paraspinal or epidural disease. Stage IV includes central nervous system and/or bone marrow involvement, regardless of other sites of involvement. Residual masses are less frequent in NHL than in HL but more frequently correspond to a persistent active disease [22].
Role of Nuclear Medicine Techniques
67Ga-citrate scintigraphy and bone scintigraphy have been replaced by [18F]FDG-PET/CT. Compared to 67Ga-citrate scintigraphy, [18F]FDG-PET detects more disease sites [27, 28], especially in the abdomen, where gallium scintigraphy is limited by physiologic bowel uptake. The other advantage of [18F]FDG-PET over 67Ga-citrate scintigraphy is same-day imaging with PET (in contrast with imaging over 2 or 3 days for gallium) and a more favorable dosimetry [28]. Bone scintigraphy is also less sensitive than [18F]FDG-PET to detect bone and bone marrow involvement [29].
[18F]FDG-PET/CT
The majority of PET systems now consist of hybrid PET/CT scanners which use the CT portion of the examination for attenuation correction and anatomic localization of lesions identified on PET. Low-dose CT (typically 5 ma at 80 kVp for attenuation correction/anatomic correlation, which may be increased to several hundred mA for thin slice diagnostic quality contrast-enhanced CT) that is usually performed has been shown to be more accurate in adults with lymphoma than PET alone [30]. However, contrast-enhanced diagnostic CT (ce-CT) remains mandatory at least at diagnosis (performed either simultaneously with PET or separately).
Diagnostic CT reliably detects enlarged lymph nodes, and contrast media are required to accurately distinguish lymphadenopathy from other structures, especially in the abdomen and pelvis due to the lack of retroperitoneal fat in children [31]. Diagnostic CT also allows detailed evaluation of pulmonary parenchyma, pleura, and pericardium.
[18F]FDG-PET/CT must also be compared to:
Ultrasonography, which has a definite role in both initial evaluation and follow-up of superficial lymph nodes. It is also the best method to detect testicular infiltration [31] and an effective imaging method to explore liver and spleen [31].
Chest radiography, which remains useful in HL to classify disease as “bulky” or “nonbulky,” which constitutes an important prognostic factor. Disease is classified as “bulky” when the ratio of the largest tumor diameter to chest diameter is greater than one-third.
[18F]FDG-PET/CT frequently detects nodal and extranodal disease sites that are missed by conventional staging methods and improves the characterization of lesions that are equivocal on other types of imaging. [18F]FDG-PET/CT is especially accurate to detect bone marrow disease, even in the case of negative iliac crest bone marrow biopsy. Various studies in adults indicate that [18F]FDG-PET for the detection of bone marrow involvement is superior not only to conventional imaging modalities but also to bone marrow trephine biopsy [28, 35, 36]. This also appears to be true in children [28, 37–40]. When bone marrow involvement is detected by PET, it is often not detected by diagnostic CT and must be confirmed by another modality, preferably MRI or bone scan when MRI is not available. In contrast, [18F]FDG-PET/CT can understage disease, especially in the case of lung sites (more reliably detected by diagnostic CT than by PET) [40] and because some lesions, even large lesions, may not be [18F]FDG avid, while other lesions take up [18F]FDG. Careful comparison of functional and morphologic imaging therefore remains essential.
Indications for [18F]FDG-PET/CT
Only a few studies have assessed the role of [18F]FDG-PET in childhood lymphoma, mainly consisting of short series [27, 37, 38, 40–50] and review articles [28, 51, 52]. The objectives of [18F]FDG-PET/CT are different in HL and NHL and will be discussed separately.
Hodgkin’s Lymphoma
HL has a reported [18F]FDG avidity of 97–100% (defined as at least one [18F]FDG-avid HL site detected, on a per-patient basis), according to four studies, comprising a total of 489 patients (adults and children), with an unspecified proportion of children [53–56].
No difference in intensity of uptake is observed according to HL subtypes [57], and the intensity of uptake at diagnosis does not appear to have any prognostic value.
[18F]FDG-PET/CT at staging must be performed before starting chemotherapy, as [18F]FDG-PET very rapidly reflects the metabolic response to chemotherapy. In children with aggressive disease, [18F]FDG-PET must also be performed before corticosteroid therapy, as HL is a malignancy comprising a large background of inflammatory cells [58, 59].
Initial Staging
In adults, [18F]FDG-PET/CT is useful for pretreatment staging. PET/CT cannot replace diagnostic ce-CT or bone marrow biopsy, but it can provide complementary information, potentially resulting in a modification of disease stage (usually upstaging) in about 15–20% of adult patients, with an impact on management in about 5–15% [60]. In children, [18F]FDG-PET modified the stage in 10–23% of patients [28] (Fig. 25.1). Apart from its role in staging and radiation therapy planning (all involved sites must be irradiated), pretreatment [18F]FDG-PET/CT is also essential because it provides a reference, facilitating interpretation of subsequent treatment evaluation PET, as recurrences usually occur in previously involved lesions, and de novo sites of uptake observed during follow-up must be primarily suspected to be false-positive [61].
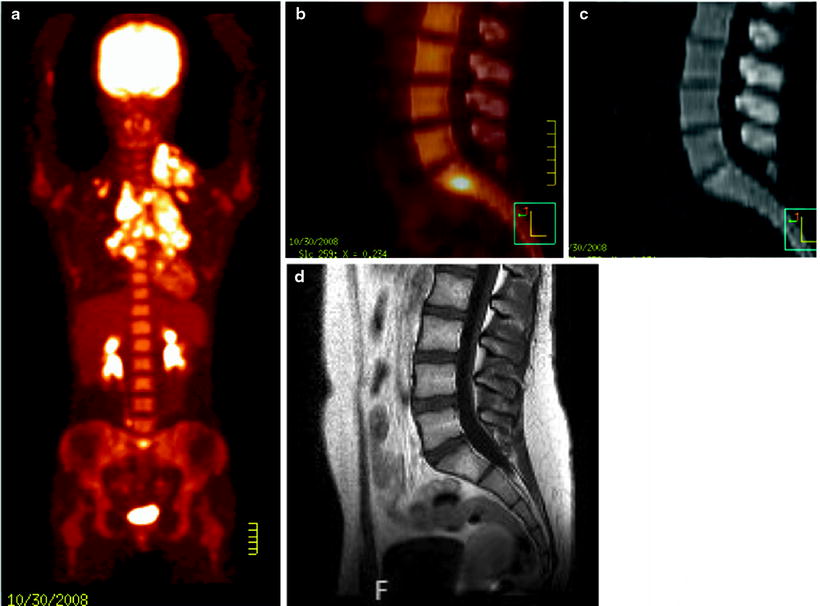
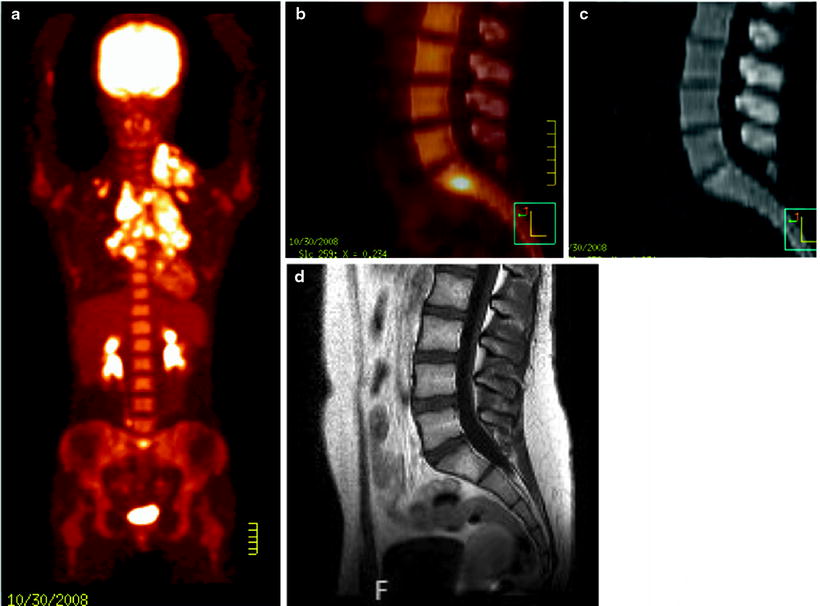
Fig. 25.1
Hodgkin’s lymphoma in a 14-year-old girl, classified stage II before [18F]FDG-PET/CT with extensive supradiaphragmatic lymph node involvement. [18F]FDG-PET/CT showed an unexpected focus in the sacral bone marrow (a and b), with no abnormality on CT (c). Bone marrow involvement was confirmed by MRI (d), leading to upstaging of the disease from stage II to stage IV
Monitoring of Response to Therapy
As HL is a curable disease, it is essential to ensure that therapy has been fully effective at the end of treatment. [18F]FDG-PET/CT is routinely performed for this purpose, usually after completion of chemotherapy and before radiotherapy, as negative [18F]FDG-PET is a reliable indicator of complete remission [60] (Fig. 25.2). The metabolic information provided by [18F]FDG-PET/CT at the end of therapy is very important, as assessment of response by morphologic imaging modalities is particularly difficult, due to the generally slow and protracted regression of the size of enlarged lymph nodes [28]. Moreover, in HL, malignant cells comprise only a small fraction of the tumor volume, which is mostly composed of reactive infiltrating cells not directly affected by antineoplastic therapy [62].
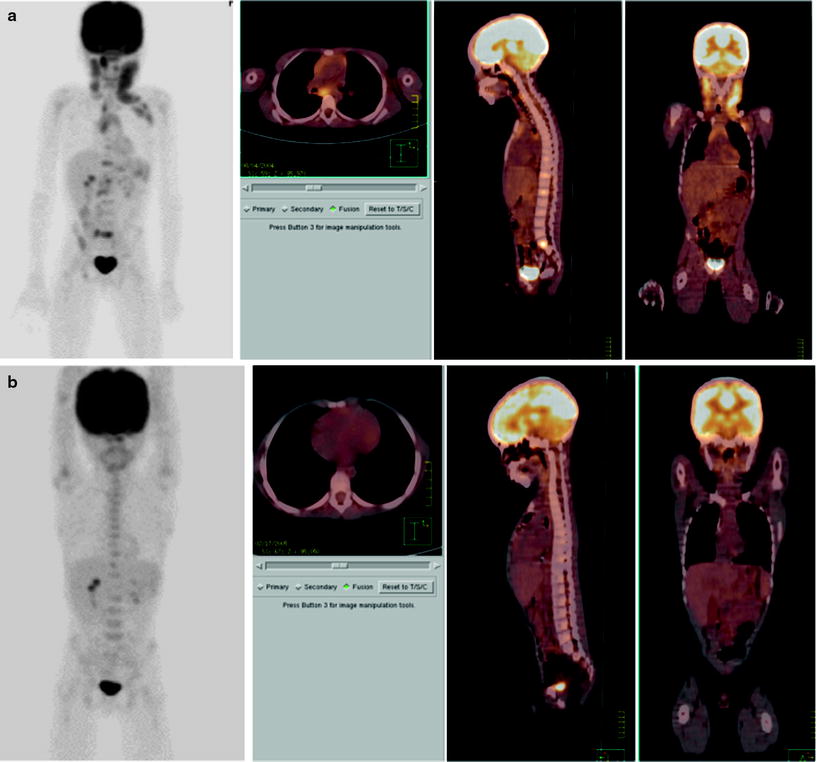
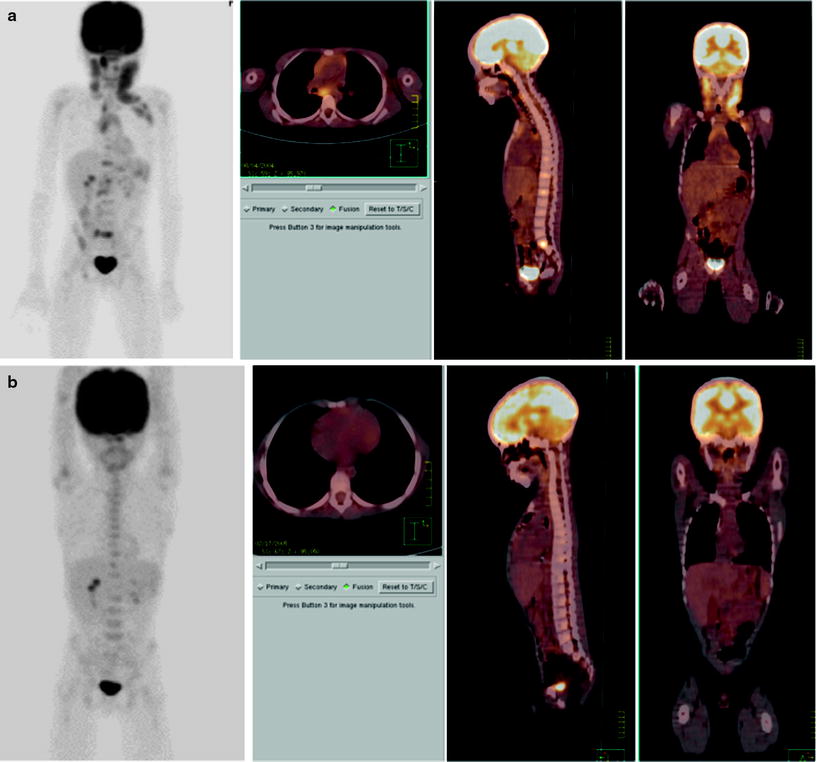
Fig. 25.2
Hodgkin’s lymphoma stage IV (lymph node and bone marrow involvement) in an 8-year-old boy. [18F]FDG-PET/CT at staging (a) and after completion of chemotherapy and before radiotherapy (b), with metabolic complete response. Persistent clinical remission 4 years after the end of therapy
Early response assessment can also be evaluated by [18F]FDG, as PET has been shown to be an effective prognostic tool in adults with HL [62]. Interim PET must not modify the scheduled therapy except in the context of a controlled clinical trial. When interim PET is negative, no other examination needs to be performed at the end of therapy in the absence of clinical signs [63]. Systematic [18F]FDG-PET is not indicated during follow-up due to the risk of false-positive results [64] and must only be performed in the case of suspected recurrence.
Non-Hodgkin’s Lymphoma
In contrast with HL, [18F]FDG uptake cannot be considered to be constant in NHL. In adult NHL, [18F]FDG uptake tends to be more intense in higher-grade lymphomas than in lower-grade lymphomas [65] and some low-grade NHL, especially T-cell lymphoma, present inconstant [18F]FDG uptake [53]. As most NHLs in children are high-grade and aggressive tumors, it can be assumed that false-negative PET at staging would probably be very rare. However, no study has specifically assessed [18F]FDG uptake according to the various subtypes of NHL in children. According to 3 studies [53–55], based on a mixed population of adults and children, [18F]FDG avidity (defined as at least one [18F]FDG-avid lymphoma site detected on a per-patient basis) was 100% for Burkitt’s lymphoma (24/24), 97% for large B-cell lymphoma (294/303), 100% for lymphoblastic lymphoma (6/6), and 100% for anaplastic large-cell lymphoma (24/24), corresponding to the most frequent subtypes of NHL encountered in children. However, in a limited experience of [18F]FDG-PET performed for NHL in 18 children, we reported 2 false-negative [18F]FDG-PET in patients with anaplastic T-small cell lymphoma, an uncommon subtype of anaplastic T-cell lymphoma [66].
[18F]FDG-PET staging is frequently difficult to organize and rarely performed due to the urgency of therapy for NHL in children. Moreover, and in contrast with HL, in which all disease sites must be precisely detected in order to plan therapy, the detection of all involved sites is less important in NHL, as treatment consists of high-dose intensive chemotherapy without radiotherapy. [18F]FDG-PET is therefore rarely performed at staging in NHL and is not performed routinely during follow-up.
The main indications for [18F]FDG-PET in children with NHL are inconclusive results of conventional imaging modalities, that is, suspicion of recurrence (Fig. 25.3) or characterization of residual masses. [18F]FDG-PET performed for these purposes can be difficult to interpret firstly because a reference [18F]FDG-PET is usually not available for comparison (and there is no absolute certainty that the NHL subtype is able to take up [18F]FDG). [18F]FDG-PET can also be falsely positive in the presence of inflammation and/or necrosis, very frequent after surgery and chemotherapy for gut involvement in Burkitt’s lymphoma [67].
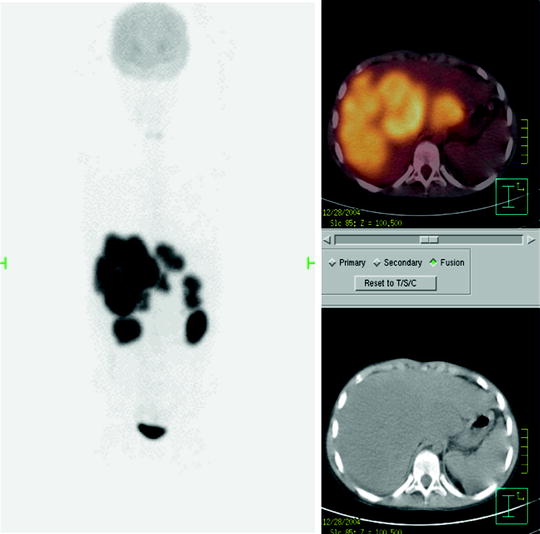
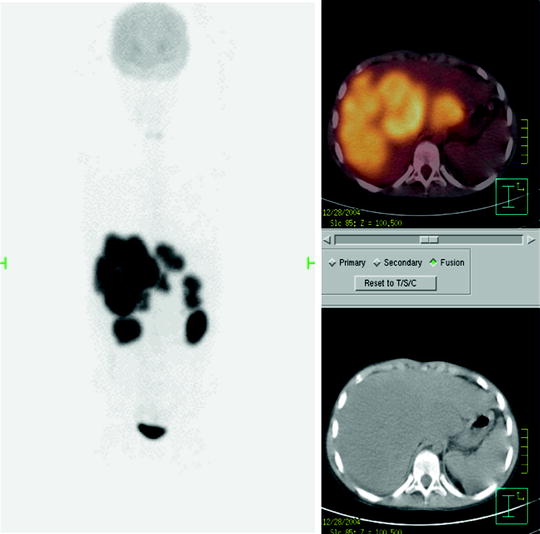
Fig. 25.3
Liver recurrence of Burkitt’s NHL. Note the very high intensity of [18F]FDG uptake by liver lesions, higher than physiologic brain uptake
However, [18F]FDG-PET appears to be a useful tool for characterization of residual masses, as no reliable CT or MRI criteria are available for distinguishing residual disease from fibrosis or necrosis [68]. In the presence of a posttreatment residual mass, negative [18F]FDG-PET has been shown to be a reliable indicator of complete clinical remission [28].
Limitations of [18F]FDG-PET
False-Negative Results
It must be remembered that, even with the most recent PET scanners, [18F]FDG-PET is unable to detect low-grade metabolic lesions measuring less than about 5 mm. Very small lung nodules, with minimal increased metabolic rates, are therefore more reliably detected by diagnostic CT than by PET [40]. During follow-up, persistence of microscopic active disease within residual masses also cannot be detected by PET. Hyperglycemia may also induce false-negative results by reducing [18F]FDG uptake in the tumor [28].
Physiologic Uptake, Pitfalls, and False-Positive Results
Certain physiologic sites of [18F]FDG uptake in children must be recognized. For example, physiologic [18F]FDG uptake may be observed in the endometrium (during menstruations) and in the ovaries (usually at mid-cycle) in adolescent girls and should not be confused with residual or recurrent tumor in pelvic lymph nodes [69, 70]. Due to the increasing resolution of PET systems, some normal structures can now appear to be slightly active on PET, for example, spinal cord, especially in the 2 sites of physiologic anatomic enlargement, that is, cervical and lumbar regions [71].
In children thymus uptake of [18F]FDG frequent, physiologic and enhanced by treatment. The physiologic appearance of thymus uptake is usually easily recognized because it is diffuse and moderately active and frequently has an inverted “V” shape. Sometimes, especially in the presence of an accessory gland, differentiation of normal thymic activity from tumor or inflammation may be difficult or impossible, and PET must then be compared with MRI, which is able to differentiate thymic tissue from recurrent lymphoma [28]. Characterization of the mass can be particularly difficult when ectopic thymic tissue is located in the neck [72].
Brown fat activation is also frequently seen in children. Brown fat is normally distributed in the neck, around the diaphragm, in the perinephric space, and in para-aortic and intercostal regions [69]. Brown fat activation is more easily recognized with hybrid PET/CT scanners (Fig. 25.4), as [18F]FDG uptake corresponds to low-density structures on CT. Even after comparison with CT images, interpretation remains difficult when brown fat activation occurs in previously involved sites. Brown fat activation can be prevented by keeping children warm prior to [18F]FDG administration and during uptake, but these measures may not be sufficient. Benzodiazepine pretreatment may also reduce this activation [68]. More recently, beta-blockers have been shown to be particularly effective and can be used with the agreement of pediatric oncologists [73].
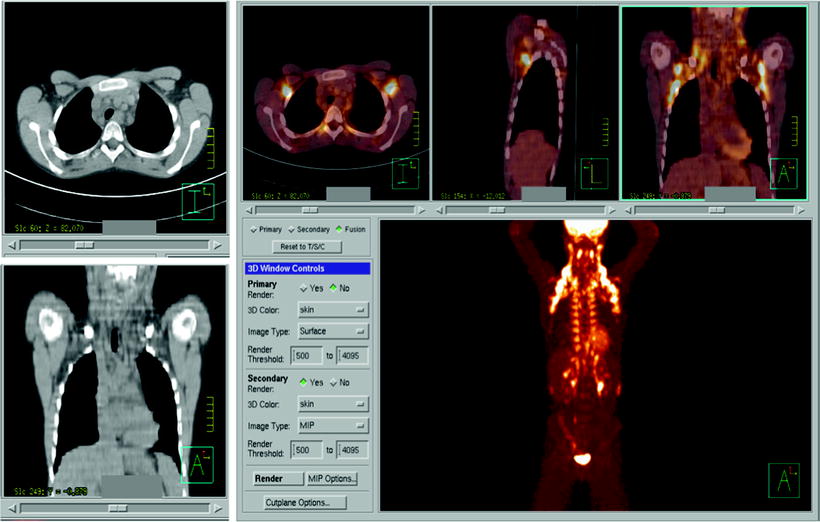
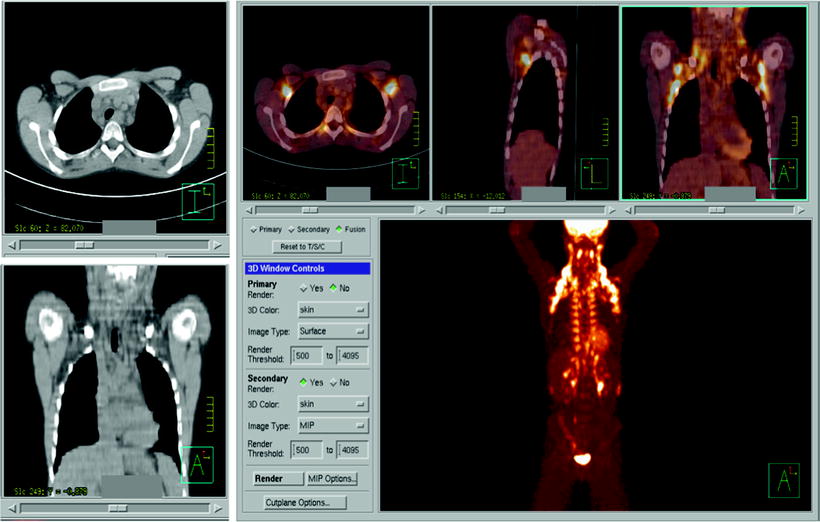
Fig. 25.4
Extensive brown fat activation. Correlative [18F]FDG PET and CT imaging easily identifies the low density of fat on CT images
False-positive results are relatively frequent because [18F]FDG is not specific for malignancy and increased uptake can occur in benign conditions associated with increased glycolysis, such as infection, inflammation, granulomatous disease, and more rarely benign tumors (e.g., Fig. 25.5).
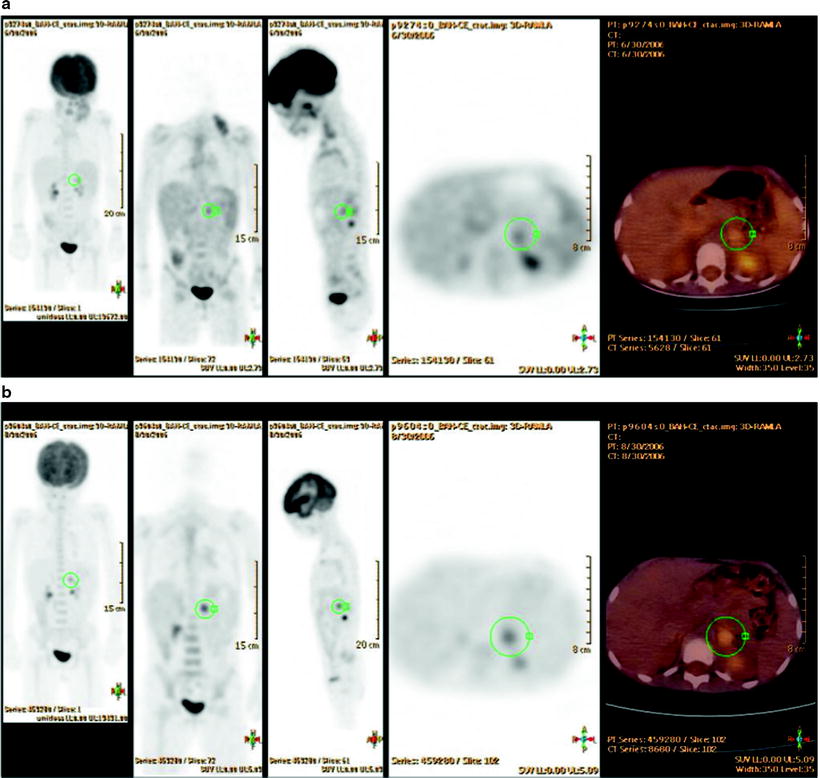
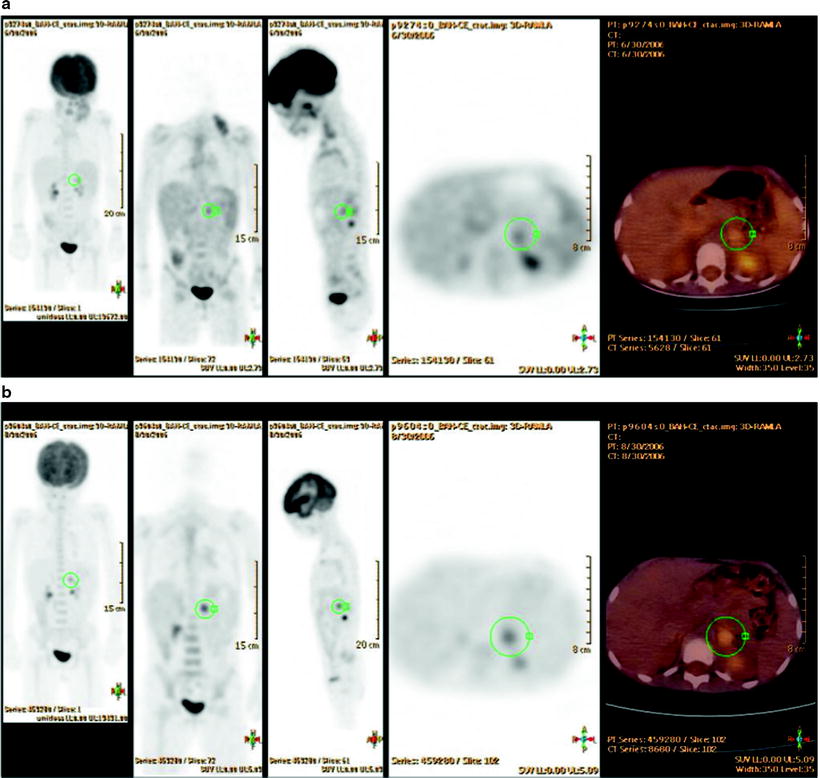
Fig. 25.5
Unexpected [18F]FDG uptake in the left adrenal gland in a 5-year-old girl with stage II HL (supradiaphragmatic lymph node involvement), at staging (a) and persistent after chemotherapy (b) although all supradiaphragmatic sites became [18F]FDG negative. This adrenal uptake was suspected to be a false-positive finding, which was confirmed at surgery. The adrenal lesion was benign, corresponding to a leiomyoma
False-Positive Results Can Also Be Treatment-Induced
In patients treated with low-dose, involved field radiation, [18F]FDG uptake can be slightly increased even months after therapy [69], but the uptake observed in a context of acute radiation pneumonitis can be intense [69].
Granulocyte colony-stimulating factor (GCSF) is a useful adjunct in patients receiving myelosuppressive chemotherapy. Administration of hematopoietic growth factors can induce reconversion from fatty marrow to red marrow, resulting in increased [18F]FDG uptake in the spine, pelvis, and long bones and in the spleen [69]. Increased activity in the spleen has also been observed both during and after GCSF treatment [69]. However, this very diffuse and homogeneous uptake is usually easy to recognize and typically declines after discontinuation of treatment but can remain increased for up to 4 weeks post-therapy [69].
Prospects
[18F]FDG-PET/CT is already an integral part of the management of childhood HL for staging, adaptation of conformal radiotherapy, and assessment of cure and suspected recurrence. [18F]FDG-PET/CT is the subject of a major ongoing European study (Euronet) designed to minimize late toxicity of therapy without compromising the cure rate. Patients who obtain a good early response to two cycles of chemotherapy (complete remission or partial remission and negative [18F]FDG-PET) do not receive radiotherapy [28].
The role of [18F]FDG PET in childhood NHL needs to be defined in controlled trials designed to address several major issues: Does NHL histology affect PET staging and PET response? What is the optimal timing of interim PET during NHL treatment? Should children with poor early PET be considered for treatment intensification? [28].
Wilms’ Tumor
Wilms’ tumor is the most common renal malignancy in childhood [76]. The five-year survival rate currently is about 91.9% [77]. Therapy follows multimodality approaches, including surgery/nephrectomy, chemotherapy, and radiation treatment. In general, recurrent disease manifests as local relapse, in the lungs, regional lymph nodes, distal urinary tract, and liver, but may also occur elsewhere [76]. Two histopathologic findings are described: the favorable and the anaplastic.
Imaging studies are mainly used to define the origin and anatomic extent of the tumor, determine the status of metastatic disease, survey therapy, and detect relapse. So far, the diagnostic workup of newly diagnosed Wilms’ tumor includes a CT or MRI scan of the abdomen and pelvis, lymph nodes, and intra-abdominal or pelvic tumor deposits. A Doppler ultrasound is recommended to evaluate if there is a tumor thrombus in the renal vein and inferior vena cava [76]. For detection of bilateral disease and the assessment of nephroblastomatosis representing premalignant lesions, MRI is the imaging modality of choice (Fig. 25.6) [78]. In cases with unclear definition of the organ of origin (kidney, adrenal gland, sympathetic trunk), 123I-MIBG scintigraphy can be very helpful for the differentiation of neuroblastoma versus Wilms’ tumor (Fig. 25.7).
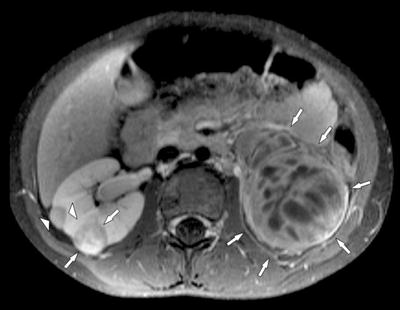
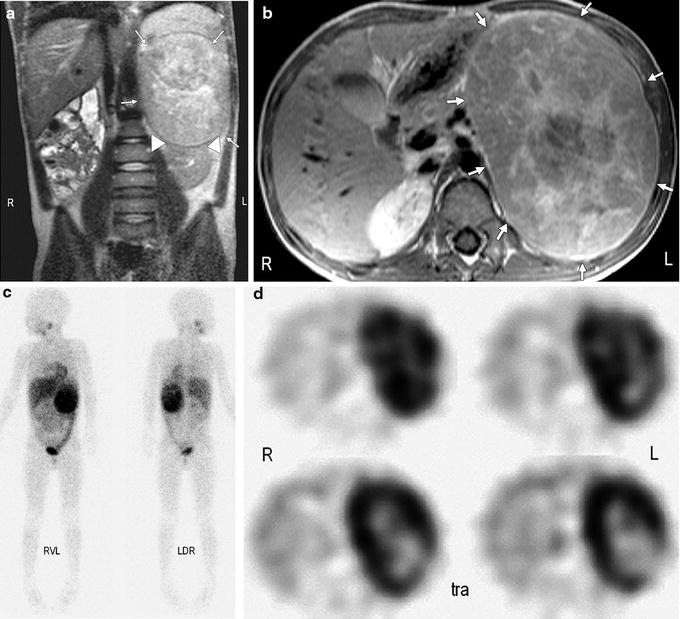
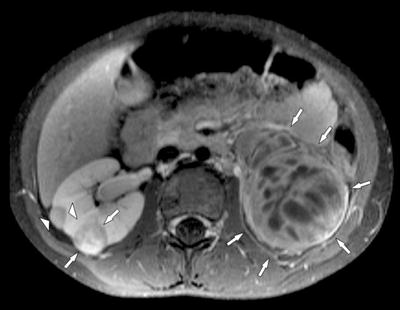
Fig. 25.6
Four-year-old boy with bilateral Wilms’ tumor and nephroblastomatosis. Transversal fat-suppressed T1-weighted image after contrast enhancement reveals bilateral nephroblastoma (arrows) and a right-sided nephroblastomatosis lesion (arrowheads)
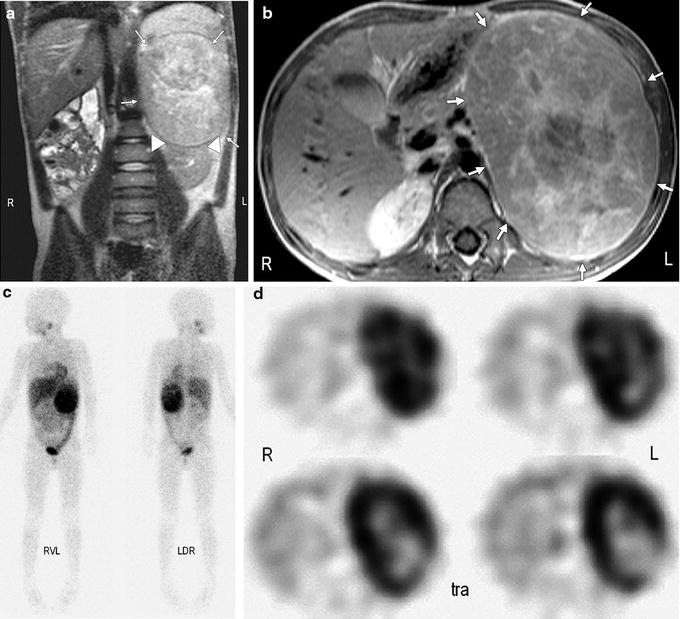
Fig. 25.7
Six-year-old boy with large neuroblastoma originating from left adrenal gland. (a) Coronal T2-weighted image reveals large mass in left abdomen (arrows) with appearance typical of Wilms’ tumor with pseudocapsule and apparent origin from kidney (arrowheads). (b) Corresponding transverse T1-weighted image depicts large mass (arrows). (c) Strong focal uptake by mass visible on 123I-MIBG scintigrams led to correct differential diagnosis of neuroblastoma. RVL = right side, ventral view, left side; LDR = left side, dorsal view, right side. (d) Transverse (tra) SPECT reconstructions that correlate to MRIs show tumor extent and central tumor necrosis
Chemotherapy is usually used prior to surgery. Therefore, a reliable response assessment using noninvasive imaging even prior to definite surgery is needed to modify therapy and to gain prognostic information [79].
Although [18F]FDG-PET was successfully used to image Wilms’ tumor for the first time [79] in the 1990s, and [18F]FDG uptake is usually consistent with a metabolically active tumor, no difference in uptake can distinguish between favorable and unfavorable histological findings [76]. Only a few years later, it became known that [18F]FDG-PET did not provide additional information during the first diagnostic workup but appeared helpful in the follow-up [79].
PET/CT scans of patients with extended disease were studied in a follow-up setting. [18F]FDG-PET was reported to detect metastatic lesions that would have been missed by the conventional imaging [76–80]. Nevertheless, [18F]FDG-PET failed to visualize small pulmonary metastases [76]. Consequently, PET/CT with the increased spatial resolution of the CT component seems to be the modality of choice in defining tumor involvement and evaluating therapy in patients with extended disease, in particular for tumors that are not amenable to surgical resection [76]. Whether PET/CT is useful for primary diagnosis of Wilms’ tumors is yet to be determined. Therefore, [18F]FDG-PET/CT does not seem to be of great importance for staging patients with Wilms’ tumor with limited disease [79].
Neuroblastoma
Neuroblastoma, an embryonic tumor, is the most frequent extracranial solid malignancy in pediatric patients (about 8% of pediatric malignancies) and remains, despite treatment intensification, responsible for approximately 15% of cancer deaths in children [81–84]. It arises from the neural crest cells, which form the adrenal medulla and the sympathetic nervous system [85]. The tumor is usually situated in the adrenal gland or anywhere else along the sympathetic nervous system chain [81]. At diagnosis, roughly 50% of patients have distant hematogenous metastases [86]. Neuroblastoma presents a great heterogeneity concerning clinical behavior and survival rates; therefore, staging is crucial in order to choose the appropriate treatment [87].
The therapeutic spectrum depends upon clinical stage and consists of supportive care with no treatment (stage IVS), definitive excision if possible, or chemotherapy before and after surgery. The treatment approaches are partially combined with total-body irradiation and 131I-MIBG therapy, followed by autologous bone marrow transplantation [84, 86, 88, 89].
Imaging of neuroblastoma consists of sonography, computed tomography (CT), MRI, and radionuclide examinations such as scintigraphic bone scanning, 123I-MIBG scintigraphy, positron-emission tomography (PET) with different tracers (primarily [18F]FDG), and recently hybrid imaging (PET/CT and SPECT/CT) [90–101].
MIBG Scintigraphy
Radiolabeled MIBG was originally developed in the late 1970s as a norepinephrine (NE) analog for imaging of the adrenal medulla [102, 103]. With the amount of radioiodinated MIBG administered for diagnostic imaging, uptake is mediated primarily by the NE transporter located on the surface of sympathetic neurons [104, 105]. Based on this mechanism, both 123I-MIBG and 131I-MIBG, have proven effective agents for scintigraphic imaging of tumors arising from the embryonologic precursors of the sympathetic nervous system, particularly the neural crest tumors neuroblastoma and pheochromocytoma [106, 107].
Scintigraphy with 123I- or 131I-labeled MIBG has become a well-established method in the diagnosis and staging of neuroblastoma [108] because of its high specificity, which is reported in the literature to be between 90% and 100% [88, 109, 110]. For diagnosis, 123I-labeled MIBG is superior to 131I-MIBG [111]. Due to more favorable dosimetric properties, a higher activity of 123I-MIBG can be administered enabling to obtain SPECT acquisitions that may increase sensitivity and provide more precise anatomical localization of the disease especially after coregistration with radiological modalities such as CT scan or MRI [112].
Reasons for false-negative studies are not entirely clear: it may be related to modifications of the active uptake mechanism due to differentiation of tumor cells or to pharmacologic interference [113–115]. Pharmacological interference (see footnote listing interfering substances)1 is probably the most frequent cause of a false-negative study. False-negative MIBG findings have been described in children with ganglioneuroblastoma, where the ganglioneuroma elements were predominant, suggesting that uptake is influenced by histology and the degree of tumor cell maturation, whereas no relationship with the secretion of catecholamines could be assessed [113]. However, other studies in children with complete negative MIBG scans at diagnosis did not find any correlation with histopathology, biological factors or stage of disease [108].
When looking at lesion-based evaluations, bone and bone marrow lesions are identified more consistently than soft-tissue lesions. Overall sensitivities range between 60% and 70% [109, 116, 117].
Physiological distribution of MIBG is resent in the heart and salivary glands because of their sympathetic innervation, while other systems (urinary tract, gastrointestinal system) represent excretion routes of the tracer [118]. Most of false-positive MIBG findings are due to a nonspecific radioactive accumulation in urinary tract, and/or gastrointestinal structures, and not to specific MIBG uptake by nonneuroblastic cells [117, 119–126]. Using SPECT/CT to record MIBG images reduces the likelihood of false-positive scans.
Bone Scintigraphy
In the literature, usefulness of bone scintigraphy for staging of neuroblastoma is under discussion. Comparing MIBG and bone scintigraphy in patients with confirmed neuroblastoma, it was found that 99mTc-MDP and MIBG scans were concordant for the presence or absence of skeletal disease. However, nearly twofold greater number of skeletal lesions was evident on MIBG scanning. In patients with histological evidence of bone marrow involvement, each study suggested skeletal lesions in approximately 70%. In addition, no patients with normal bone scans had MIBG studies indicating bone involvement. In conclusion, both, MIBG and 99mTc-MDP, are useful for the detection of skeletal neuroblastoma. 123I-MIBG is the better agent for characterizing the extent of disease, and 99mTc-MDP is a valuable adjunctive agent that provides skeletal landmarks for comparison [127].
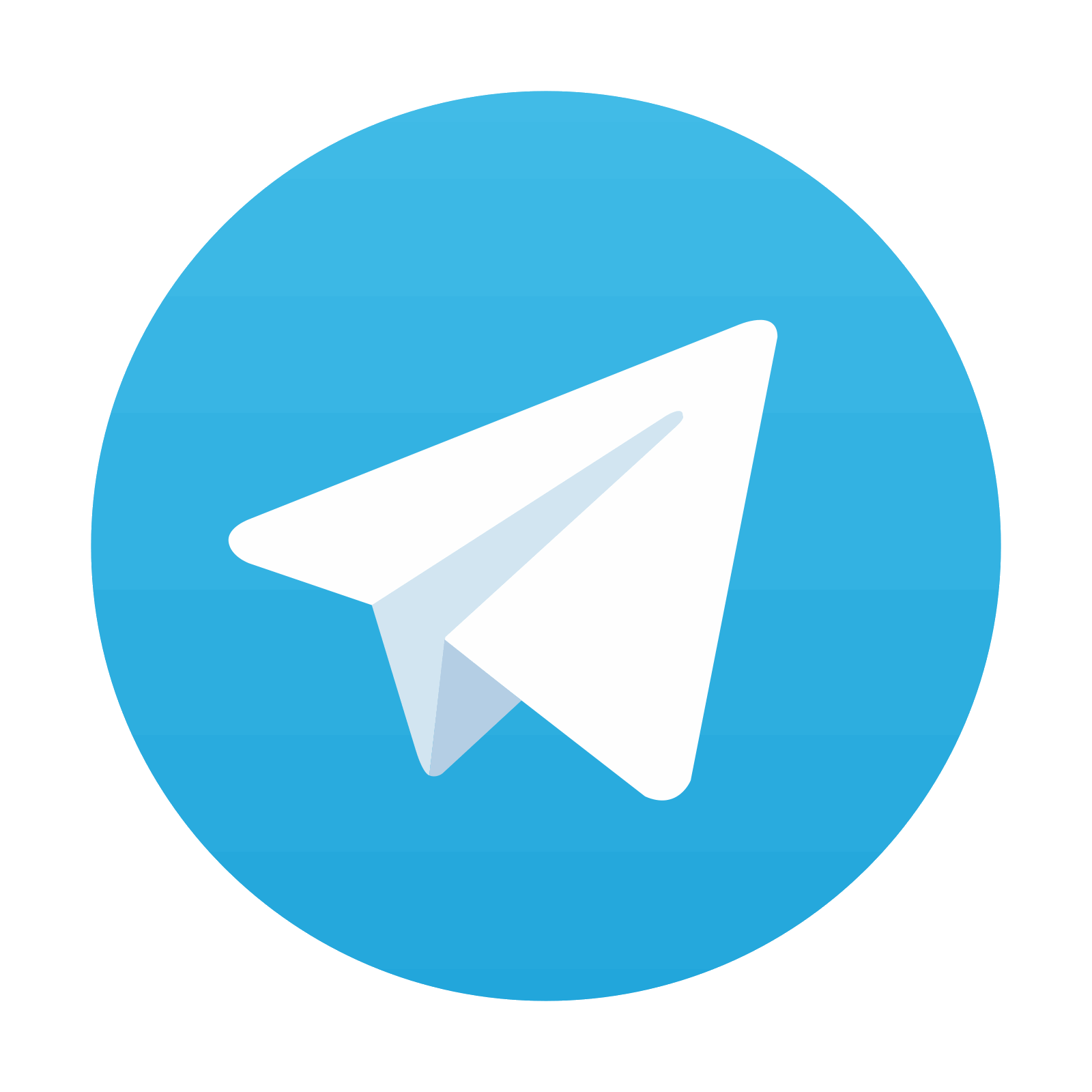
Stay updated, free articles. Join our Telegram channel
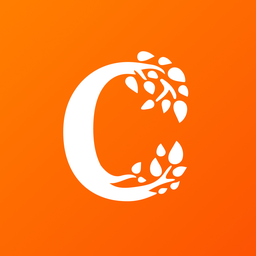
Full access? Get Clinical Tree
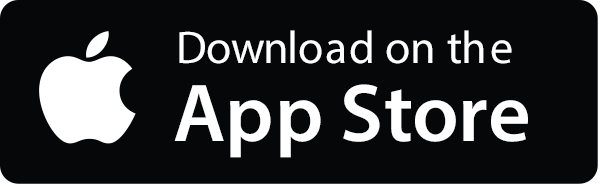
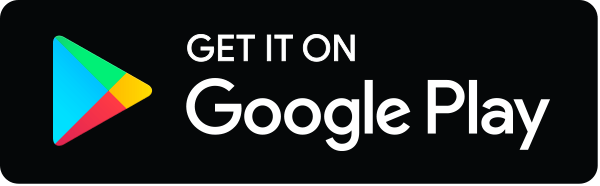