Fig. 6.1
Conventional dose-rate effect for cell killing as the dose rate is lowered from 150 to 1.6 cGy/min. The dashed curves represent the best fit to the data set obtained using the lethal–potentially lethal (LPL) model assuming either full repair (A) or no repair (B), respectively
At very LDRs (≤2 cGy/min), cell proliferation can occur during the irradiation, thus leading to repopulation of the pool of clonogenic cells. Clearly, based on this assumption RNT should be noneffective, although this expectation is not consistent with many clinical observations. Such phenomenon, in which decreasing the dose rate results in increased cell killing, has been defined “inverse dose-rate effect.” The effect has been attributed both to the lower dose rate (permitting the cells to progress through the cell cycle into more radiosensitive phases) and to some hypersensitivity of cells to low dose/LDR exposures, as will be discussed below [3, 5].
Cell Death Mechanisms
Most cellular responses to ionizing radiation-induced DNA damage are genetically regulated and involve specialized DNA damage-recognition factors that trigger a cascade of signaling events that alter the expression and/or activity of specific genes/proteins involved in cell-cycle arrest, DNA repair, accelerated senescence, and apoptosis at the cellular level and in tissue repair at the tissue level [5].
Necrosis, a form of cell death that has been variously described as generalized, nonspecific, accidental, or passive (i.e., it is not a genetically regulated process), generally occurs after high doses in cells that enter mitosis carrying high levels of unrepaired DNA damage [5].
In contrast, apoptosis is an energy-dependent, genetically controlled “suicide” process that involves the activation of degradative proteolytic enzymes called caspases and tends to occur at low doses of radiation. Apoptotic postirradiation cell death is mediated by two pathways, both resulting in activation of the cascade of caspases that function as cell executioners. Firstly, signals from the nucleus or cell membrane can activate the “intrinsic” pathway of apoptosis with a series of sequential events that cause final activation of caspases 3,6,7, and 8. Secondly, the “extrinsic” pathway of apoptosis is mediated by death receptors that are activated by ligands, such as the Fas ligand, tumor necrosis factor-α (TNF-α), and TNF-related apoptosis-inducing ligand (TRAIL). TRAIL induces apoptosis in response to ionizing radiation through the clustering of DR4 and DR5 receptors in the cell membrane [7].
Other modes of cell death are the “accelerated” or “premature” senescence or “STASIS” (stress or aberrant signaling-induced senescence), a genetically programmed response to DNA damage [8], and the mitotic catastrophe, generally defined as the failure of a cell to properly undergo mitosis after DNA damage. It has recently been recognized that mitotic catastrophe is not a mode of cell death per se, but a death that generally occurs secondarily to mitotic catastrophe, apoptosis, or accelerated senescence [9]. Death secondary to mitotic catastrophe has been suggested to account for a significant proportion of the cytotoxicity observed after radiation exposure.
Possible Contributing Mechanisms to RNT Tumor Responses
Low Dose/Dose Rate Apoptosis
There is an emerging body of evidence to suggest that different mechanisms of cytotoxicity operate in different dose and dose-rate ranges for a given cell type. Indeed, several experimental studies support the tenet that LDR exposures tend to promote loss of clonogenic potential in some cell types (e.g., lymphomas) by activating apoptotic responses, whereas high doses tend to cause necrosis as their primary mechanism of cytotoxicity [10]. For example, it has been reported that low doses of EBRT induce substantial apoptosis in tumor cells and that the dose–response curve for apoptosis plateaues above ∼7.5 Gy [11]. Furthermore, multiple small fractions of EBRT were found to produce a higher level of apoptosis than a large single dose. Clinical effectiveness of lower doses of RNT and other LDR therapies could be related to their ability to optimize such effects, apoptosis exhibiting an inverse dose-rate effect [5].
Low Dose Hyper-Radiosensitivity–Increased Radioresistance
Many cell types exhibit an initial hypersensitive response at doses below ∼25 cGy, followed by a region of increasing radioresistance up to ∼50 cGy, the survival curve above 1 Gy closely following the expected LQ response [12]. This phenomenon, illustrated in Fig. 6.2, has become known as the “low dose hyper-radiosensitivity–increased radioresistance” (LDH–IRR) response. The mechanism of LDH–IRR probably involves an alteration in the cellular processing of DNA damage as a function of dose. One possibility is that the transition from LDH to IRR may involve the activation of radioprotective DNA-repair pathways that are triggered by a certain threshold level of DNA damage. Cells would then be hypersensitive to low doses of radiation that produce insufficient DNA damage to trigger this protective process.
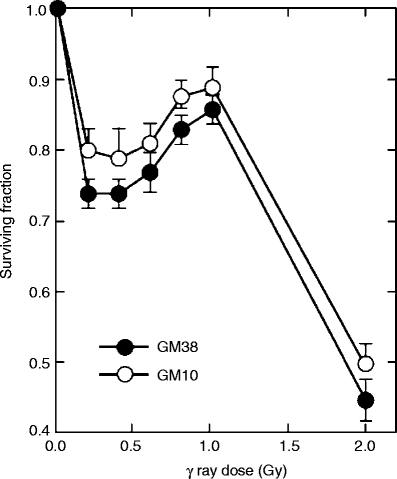
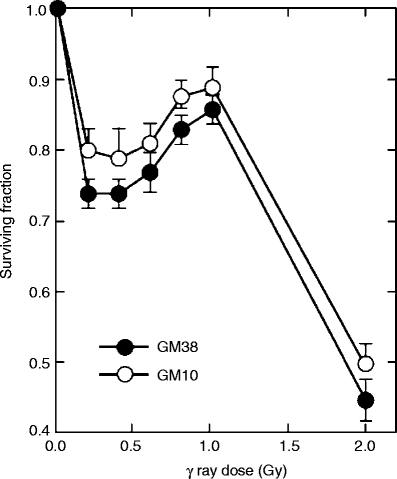
Fig. 6.2
Cellular survival responses of two normal human fibroblast strains, GM38 and GM10, following exposure to 60Co γ-radiation
LDH–IRR seems to be preferential and possibly limited to a fraction of cells in the G2 phase of the cell cycle, and an important consequence of the LDH–IRR response is that the conventional LQ model greatly underestimates cellular radiosensitivity after acute low doses. This limitation was addressed by Joiner et al. [12] in a modified LQ model that incorporates the impact of an induced repair threshold.
If the LDH–IRR response occurs also during LDR therapies, the latter could exert much greater cytotoxicity per unit of absorbed dose than would be predicted by the conventional LQ model. The LDH–IRR model leads to the prediction that DNA-repair processes should be less efficient after doses below the IRR threshold (i.e., typically ≤40 cGy) if this response is related to induced DNA repair.
G2 Synchronization, Bystander and Cross-fire Effects
In some tumor cell lines, LDR exposures in the range of 10–300 cGy/h have been shown to cause the partial synchronization of cells in the G2/M phase as a result of the prolonged activation of the G2 checkpoint [13]. This effect could have two consequences for LDR therapies. Firstly, it might abrogate the competitive effects of proliferation on the elimination of clonogenic tumor cells. Secondly, it might enhance the relative effectiveness of LDR radiation by holding the cells in G2, which is a relatively radiosensitive phase of the cell cycle, a mechanism that was invoked to explain the inverse dose-rate effect seen in some cell lines at LDR.
The “bystander” effect refers to the phenomenon whereby manifestations of damage (such as cell death) are observed in cells adjacent to those that are traversed by an ionizing particle but that are not themselves “hit” [5]. This means that a cytotoxic response in the presence of the bystander effect will be greater than that predicted on the basis of classical dosimetric estimates. The biological bystander effect appears to reflect the generation of a “damage signal” emanating from irradiated cells that is communicated to the non-irradiated adjacent cells through a variety of signaling mechanisms. These include intercellular crosstalk, the release of reactive oxygen species (ROS) or of clastogenic factors, and intracellular signal transduction pathways. The contribution from these mechanisms probably varies among different model systems, leading to some controversy as to their relative importance.
The bystander effect is quite distinct from the cross–fire effect, another mechanism of RNT, in which an ionizing particle emanating from a source radionuclide in one cell deposits its energy in a distant target volume represented by a neighboring or distant cell [13]. Cross-fire effects depend predictably on the particle range and play a major role in the effectiveness of RNT using β-emitting isotopes such as those generated by 131I that have a range of millimeters in tissue. Such cross-fire effects with β-emitters is also important for therapeutic responses, because they should help to overcome the limitations imposed on RNT by heterogeneity in the distribution of the radiopharmaceutical within the tumor mass, which is especially important in solid tumor.
Adaptive Responses
The in vitro adaptive response is the phenomenon whereby exposure of cells to a low “priming” dose of radiation induces resistance to a subsequent higher-dose exposure [14]. Like LDH–IRR, the adaptive response has been suggested to involve inducible radioprotective mechanisms, such as DNA-repair pathways, although not all studies are consistent with such a mechanism. A possible explanation for these diverse findings is the broad range of cell types, assays, priming treatments, and times of observation employed in different studies; furthermore, adaptive responses typically occur only within a narrow dose range of ∼0.5–20 cGy. With respect to the potential role of adaptive responses in RNT, it has been noted that LDR irradiation can be regarded as a series of priming doses briefly separated in time; the adaptive response could, therefore, have a negative influence on the efficacy of LDR therapies, unless, of course, it is not activated under these conditions.
Fractionated RNT and Hypoxia
Fractionated delivery of RNT is designed mostly to compensate for the anticipated heterogeneity of RNT dose distribution, which determines absent or suboptimal intratumoral radionuclide deposition, an event that is especially important in large, poorly vascularized tumors that contain regions of hypoxia [10]. The development of hypoxia in tumors is believed to represent a major barrier to successful EBRT, in part because hypoxic cells are ∼threefold resistant to acute exposures to ionizing radiation with respect to normoxygenated cells [15]. Conventional dose fractionation partially overcomes the negative effect of hypoxia, by allowing for the reoxygenation of hypoxic cells between fractions, and it is reasonable to assume that the same would be true for protracted LDR therapies. Under some conditions, fractionated delivery of radiolabeled antibodies and peptides has shown efficacy, with fractionated delivery causing less toxicity than a single administration. In general, both preclinical models and clinical radioimmunotherapy evidence suggest that RNT fractionation results in a beneficial effect and in a more uniform radiation dose distribution. This has certainly proved to be an effective strategy with [131I]meta-iodobenzylguanidine (131I-mIBG), with obvious increases in clinical effectiveness, and there are now data to support the use of fractionated treatment with radiopeptides as a way of reducing toxicity.
Future Directions
There is no doubt that a better understanding of the radiobiology and mechanisms of action of RNT will facilitate the development of newer radiopharmaceuticals and the design of prospective phase III and IV clinical trials [5]. Although our understanding of the low-dose-related phenomena has increased dramatically in the last few years, it is important to validate these effects in the clinical setting. In particular, validation of a role for the bystander effect in RNT in vivo would greatly impact on the way we would assess therapeutic response to RNT, as well as risk estimates for therapeutic and diagnostic radiopharmaceuticals. In addition, an area that has yet to be widely applied to LDR therapeutics concerns the application of high-throughput genomic/proteomic screening methods (DNA arrays, single nucleotide polymorphism analysis, and protein arrays) for the rapid and accurate prediction of patient response, both in the tumor and in normal tissues [5]. Other issues, such as the role of metabolic markers in selecting therapies and dosage schedules, and the potential interactions between low-dose chemotherapy and RNT constitute additional important areas for clinical development.
Dosimetry: Overview on Methods
The basic goal of RNT is to ensure that enough radiation absorbed dose is delivered to the tumor while minimizing the risk of toxicity to the bone marrow and to other normal tissues.
For RNT, many physicians administer approximately the same activity to all patients, while ideally administered activity should be adjusted using a patient-specific treatment planning strategy based on radiation absorbed dose. With this approach, activity administration is optimized to maximize treatment efficacy not delivering a radiation dose that could cause deleterious side effects to normal organs.
In RNT dosimetry, the absorbed dose is the energy deposited (E) per unit mass of matter (m) (with units of J/kg, 1 J/kg = 1 Gy). In RNT, E is the number of radionuclide disintegrations in a particular volume multiplied by the energy emitted per disintegration of the radionuclide and the fraction of emitted energy that is absorbed by a particular mass (m).
To improve the correlation between dose and the exposure effect, other quantities must be defined, such as relative biological effectiveness (RBE), radiation weighting factors, and tissue weighting factors. This suggests that energy absorbed per unit mass does not predict response at all levels, and some other factors must be considered.
Damage to cells is due primarily to indirect effects of radiation (formation of free radicals in water, that diffuse and subsequently interact with cellular components, mostly DNA) and to some degree to direct effects (direct damage to DNA from radiation interaction). Also, different tissues and different individuals have different abilities to respond to and repair this damage. Thus, physical quantities such as the absorbed dose must be linked to radiobiological quantities to completely understand and be able to predict effects in a system.
Internal dose can be calculated by the following simple equation from the medical internal radiation dose (MIRD) Committee of the Society of Nuclear Medicine (SNM) [16, 17]:

where D is the absorbed dose in a target organ (Gy),
is the cumulated activity in source region S, Δ is the energy emitted by the radionuclide per disintegration,
is the fraction of energy emitted by the radionuclide in source region S that is absorbed in the target region, and M T is the mass of region T. Furthermore, τ is the residence time, which is simply equal to Ã/A 0, the cumulated activity divided by the patient’s administered activity (A 0). S is the absorbed dose per unit cumulated activity and it is given by
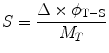

(6.1)


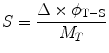
(6.2)
Equation (6.1) means that the absorbed dose depends on the half-life of the radionuclide and its spatial and temporal distribution in the target. The latters are typically obtained by images collected at different times after administration of the radiopharmaceutical and used to estimate the amount or concentration of radioactivity in a specific region. The level of activity obtained at different times after injection, plotted against time, gives a time-activity curve for a particular target, such as an organ or tissue. The integral of this curve gives the total number of disintegrations or the cumulated activity (Ã) for the region. Therefore, the main input data needed for evaluation of radiation dose are the biokinetic data that characterize the distribution and retention of the radiopharmaceutical throughout the biological system.
This absorbed dose could be used to estimate the biologic effective dose (BED) [18]:
![$$ \text{BED}=D \times \text{RE}\ \text{where} \ \ \text{RE}=\left[1+\frac{D\lambda }{(\alpha /\beta )(\mu +\lambda )}\right]$$](/wp-content/uploads/2016/08/A78639_1_En_6_Chapter_Equ3.gif)
where D is the absorbed dose, μ is the exponential repair rate constant that quantifies the rate of sublethal damage repair, and λ is the effective clearance rate constant (given by the sum of the physical decay and the biological clearance rate constants). It means that the BED may be defined as the product of the total physical dose D and a modifying factor named the “Relative Effectiveness per Unit Dose,” RE, that quantifies dose-rate effects with respect to radiosensitivity and repair of radiation damage.
![$$ \text{BED}=D \times \text{RE}\ \text{where} \ \ \text{RE}=\left[1+\frac{D\lambda }{(\alpha /\beta )(\mu +\lambda )}\right]$$](/wp-content/uploads/2016/08/A78639_1_En_6_Chapter_Equ3.gif)
(6.3)
The BED equation is derived from the linear-quadratic (LQ) model that describes the surviving fraction (S f) of target cells after a radiation dose (D):
where the linear component αD describes the DSBs induced by a single ionizing event, and the quadratic component βD 2 describes the same effect induced by two separate ionizing events; α and β are the tissue-specific coefficients for radiation damage, α being proportional to dose (one single event is lethal) and β being proportional to squared dose (two sublethal events required for lethal damage). The α/β ratio is also named “repair capacity,” and quantifies the sensitivity of a given tissue to changes in fractionation. Typical values for the α/β ratio are about 5–25 Gy for early-reacting normal tissues and tumors and about 2–5 Gy for late-responding normal tissues.
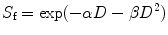
Also with the biological effective dose, the focus in radionuclide dosimetry study is to calculate the absolute amount of energy delivered per mass unit of tissue, i.e. the absorbed dose D.
In order to plan and design an appropriate dosimetric study, it is necessary to know approximately how the compound will be taken up and cleared from the various organs and the whole body, by collecting samples at the appropriate times. Most therapeutic agents have a relatively fast phase of organ uptake and initial clearance, followed by more general systemic removal that lasts for many days. Therefore, a typical sampling scheme is to collect several samples in the first hours after administration, then about once or twice a day for a few days to 2 weeks.
To calculate the cumulated activity, the integral of the time-activity curve for each source organ may be obtained by (1) direct integration of data, for example with the trapezoidal method, (2) a fit of the data to yield a mathematical expression of the uptake and retention in the target organ, (3) using compartmental models, if the pharmacokinetics inside the target tissue is available.
Specific quantification techniques have been summarized for 2D and 3D imaging in the MIRD16 and MIRD 17 [19, 20].
Using planar data (2D Imaging), the most accepted technique is to obtain images from the posterior and anterior projections, then correct the projected data in each region of interest (ROI) for attenuation and scatter. The most popular technique for attenuation correction involves the use of a Cobalt-57 (or other appropriate radionuclide) projection source imaged with and without the patient in the view, the attenuation coefficient for the system having been characterized in advance. For scatter correction, the two- or three-energy window method is widely accepted and applied when gamma camera software permits simultaneous acquisition in multiple energy windows. One of the most known packages able to offer mean absorbed doses in the organs, based on uniform activity distribution in organs/tissues was the MIRDOSE3.1 package, that implemented the use of whole-body MIRD stylized mathematical phantoms representing adult males and females, children, and pregnant women [21]. The MIRDOSE3.1 software could be used for calculating internal dose for a large number of radiopharmaceuticals, the rapid comparison of calculations for different cases, examination of dose contributions to different organs, and regional marrow dose calculations.
MIRDOSE3.1 has been updated to a new generation code, Organ Level Internal Dose Assessment (OLINDA), employing the Java programming language and the Java Development Kit environment [22]. The entire code was rewritten, but all of the basic functions of the MIRDOSE code were retained, while others were extended. More individual organ phantoms were included, the number of radionuclides was significantly increased (including alpha emitters), and the ability to perform minor patient-specific adjustments to doses reported for the standard phantoms was made possible.
Quantitative 3D imaging using single photon emission computed tomography (SPECT) methods is considerably more complex. The essential requirements for 3D imaging-based dosimetry are the availability of 3D anatomic imaging studies, such as CT or MRI, at least one 3D imaging study of the radioactivity distribution (e.g., PET or SPECT), and software that implements a point-kernel or Monte Carlo calculation methodology to estimate the spatial distribution of absorbed dose. Two fully developed packages are 3D Internal Dosimetry (3D-ID) and DOSIMG [23, 24].
Clinical Experience
Clinical applications of dosimetry are not widely adopted in RNT, mainly because data from prospective randomized clinical trials that may prove the effectiveness of dosimetry in predicting clinical outcome after treatment are lacking. In order to prove that dosimetry-based RNT is of additional benefit over administration of fixed empirical activities or activities per body weight, prospective randomized phase III trials with appropriate endpoints should be undertaken [25]. So far, the lack of standardized methodology for calculating the absorbed doses and the continued use of approaches based on administrations of fixed empirical activities irrespective of personalized radiation dose estimates has hampered efforts to compare clinical outcomes in different patient populations and has led to significant difficulty in comparing results among different trials [4].
131I-Iodide Therapy of Differentiated Thyroid Carcinoma
Radioiodide therapy has largely been proven to be a safe and effective method in the treatment of patients with DTC after total or near-total thyroidectomy [26]. Nevertheless, although it has been shown to be useful for ablation of thyroid remnants and for treatment of locoregional or distant metastases, at present there is no consensus on the activity of 131I-iodide to administer, because of the lack of prospective, randomized data [27]. Many excellent reviews of empiric fixed prescribed activity have been previously published, and the most frequently used sets of empiric fixed prescribed activities range from 1.1–5.5 GBq for ablation to 5.5–11.0 GBq for treatment of metastases [28].
The advantages of using the empiric fixed prescribed activity are convenience, a long history of use, an acceptable rate and severity of complications, and the possibility to avoid the “stunning” phenomenon due to the diagnostic 131I-iodide activity [27].
However, the persistence of disease in a significant proportion of patients and the possibility for multiple small empiric activities to have less therapeutic benefit than the same total activity given at one time has led to attempt to improve such empiric approach to therapy [28].
Efforts to meet this goal have led to two basic dosimetrically determined prescribed activity approaches, each one addressing a different aspect of this problem. Benua et al. developed an approach to define the maximum activity of radioiodide that can be administered without significant bone marrow suppression [29]. Maxon developed a method to evaluate the activity of radioiodide needed to adequately treat metastatic lymph nodes [30].
The limited bone marrow toxicity method (Benua approach) was developed at the Memorial Sloan-Kettering Cancer Center, by setting an upper limit for the radiation dose to the patient’s blood, which is considered as a surrogate for the critical organ, i.e., the red bone marrow [29].
Since radioiodide concentration is almost identical in blood as in inner organs including the red bone marrow, assessing the blood absorbed dose allows to estimate the radiation absorbed dose that will be delivered to the hematopoietic system of the individual patient during therapy [30]. A summary of the methodologies used can be found in the review article by van Nostrand [28] and in the guidelines of the Dosimetry Committee of the European Association of Nuclear Medicine (EAMN) for pre-therapeutic dosimetry [31].
The method allows to estimate the radiation dose that will be delivered to the hematopoietic system per unit activity administered to a given patient and restricts such dose to no more than 2 Gy to the blood [29, 31]. Therefore, the maximum activity for treatment is calculated as the amount of 131I-iodide that would deliver an absorbed dose of 2 Gy to the blood compartment [32].
This method requires evaluation of the kinetics of activity in the blood and the time changes of activity in the whole body, obtained preferably by conjugate views of a whole-body scan (WBS) acquired with a dual-head gamma camera equipped with high-energy collimators [28].
To evaluate the blood kinetics, five blood samples should be obtained over 1 week (e.g., at 2, 6, 24, 96, and 144 h) after administration of 131I-iodide, and the blood activity should be measured in a calibrated well counter from aliquots of blood samples [28].
The first WBS measurement obtained 2 h after tracer administration is considered to represent 100% of administered activity, while measurements are repeated at 24, 48, 72, and 96 h (or later if uptake and/or renal clearance are delayed). As an alternative to WBS conjugate-view imaging obtained with a gamma camera, measurements with an external probe can be used [28].
To calculate the whole-body activity as a function of time and circulating activity per mL of blood, the geometric mean of corresponding net counts obtained by conjugate views and all blood activities are normalized to the first data point and to the administered activity, respectively [28, 31].
The curves, A(t), describing the activity in blood and in the total body as a function of time after the administration are usually multi-exponential, and bi-exponential fitting is usually appropriate to determine the function describing time-activity curves in the blood and in the total body, respectively.
The residence times in the whole body and activity concentration in blood, t TotalBody [h] and t Blood [h], are calculated by integrating the corresponding retention function R(t) = A(t)/A 0.
According to the generally accepted MIRD formalism, the mean absorbed dose to the blood per unit administered activity is determined by the sum of contributions of blood self-irradiation and of penetrating radiation from the whole body.
The mean blood absorbed dose per unit administered tracer activity can therefore be calculated as
![$$ \frac{{\overline{D}}_{\text{Blood}}}{{A}_{0}}\left[\frac{\text{Gy}}{\text{GBq}}\right]=108\times {\tau }_{\text{Blood}}\text[h]+\text{ }\frac{0.0188}{\text{wt}[\text{kg}]}\times {\tau }_{\text{TotalBody}}\text[\text{h}]$$](/wp-content/uploads/2016/08/A78639_1_En_6_Chapter_Equb.gif)
![$$ \frac{{\overline{D}}_{\text{Blood}}}{{A}_{0}}\left[\frac{\text{Gy}}{\text{GBq}}\right]=108\times {\tau }_{\text{Blood}}\text[h]+\text{ }\frac{0.0188}{\text{wt}[\text{kg}]}\times {\tau }_{\text{TotalBody}}\text[\text{h}]$$](/wp-content/uploads/2016/08/A78639_1_En_6_Chapter_Equb.gif)
The activity to be administered for a blood absorbed dose of 2 Gy is
![$$
{A}_{\text{Adm}}\text[\text{GBq}]\text{=}\frac{\text{2}\times {\text{A}}_{\text{0}}\text[\text{Gy/GBq}]}{{\text{D ¯}}_{\text{Blood}}}
$$](/wp-content/uploads/2016/08/A78639_1_En_6_Chapter_Equc.gif)
![$$
{A}_{\text{Adm}}\text[\text{GBq}]\text{=}\frac{\text{2}\times {\text{A}}_{\text{0}}\text[\text{Gy/GBq}]}{{\text{D ¯}}_{\text{Blood}}}
$$](/wp-content/uploads/2016/08/A78639_1_En_6_Chapter_Equc.gif)
Under the conservative assumption that the activity concentrations within the hematopoietic tissue and the blood are identical [33], a red marrow-based approach for the determination of the maximum activity to be administered has been proposed [33]. Although this method seems to be accurate, no systematic clinical validation of the red marrow absorbed dose versus toxic effect has yet been undertaken.
The limited bone marrow dosimetry is easy to perform both pre-therapeutically and peri-therapeutically, thus allowing to increase the therapeutic activity for selected patients without risk of severe side effects. However, the original method might not to be applied in the presence of extended metastatic bone involvement, as the blood-based absorbed dose calculation could underestimate the absorbed dose to the red marrow. Similarly, it should be carefully applied also in the case of patients with diffuse lung micro-metastases, because the critical organ could be the lung itself instead of the hematopoietic red marrow [34, 35]. The main limitations of this method are reported in Table 6.1.
Table 6.1
Problems and difficulties of limited bone marrow dosimetry (Benua approach)
No valid clinical data or outcome rates yet exist on a benefit of the strategy |
The approach does not estimate the absorbed dose to the thyroid remnant or metastasis |
“Stunning effect” due to diagnostic activity of 131I could alter lesion biokinetics and the absorbed dose in a subsequent 131I therapy |
Increased cost and practical inconvenience |
The goal of the lesion-based dosimetry (Maxon approach) is to individualize radioiodide activity that delivers the recommended absorbed doses to ablate thyroid remnant or to treat metastatic disease while minimizing the risk to the patient [36]. These absorbed doses are traditionally considered to be ≥300 Gy to ablate thyroid remnant and ≥80 Gy to successfully treat metastatic disease [36].
In order to determine the activity required to deliver the absorbed doses, it is necessary to measure the uptake, the clearance, and the concentration of 131I contained in the identifiable thyroid remnants and/or metastases. One way to ascertain these parameters is through an analysis of selected regions of interest (ROIs) on conjugate-view gamma camera images or on SPECT images, obtained at sequential time points after administration of a tracer activity. Typically, these images should be acquired at 24, 48,72, and 96 h after tracer administration, but later time sampling might be necessary if the uptake and clearance are delayed. In addition, transmission images to correct for attenuation in the lesion area, scatter images, as well as calibration procedures, are necessary. A curve-fitting procedure is then employed to determine the assumed single-exponential half-life value and to extrapolate the curve to zero-time to determine the initial activity in the lesion [28].
Pre-therapeutic dosimetric assessments of the activity required to achieve a certain prescribed absorbed dose to a remnant or lesion are often based on adaptations of the generic MIRD equation for absorbed dose, as previously described.
where
denotes the mean absorbed dose to the remnant/lesion,
is the cumulative activity, and S is the “S factor” of the MIRD scheme depending on the lesion/remnant mass.



For dosimetry of metastases, CT, MRI, or US can be used for attenuation correction and to determine the mass, while no thoroughly validated method is yet available for ablative treatments to exactly calculate the thyroid remnant volume after surgery.
If the lesions are small, the “nodule module” of the OLINDA/EXM software might be useful to generate a spherical model of the remnant and/or tumor. Furthermore, if the size of the lesion is smaller than 5.0 mm (assuming that such small size can actually be accurately determined), then the tissue range of the beta particles can no longer be neglected in the dose calculation. This as well as other limitations of the lesion-based method are reported in Table 6.2.
Table 6.2
Problems and difficulties of lesion-based dosimetry (Maxon approach)
Not uniform absorbed doses within each lesion |
An accurate estimate of the lesion mass is not always possible |
Low uptake in lesions and, therefore, low count rates may cause statistical errors in the measurements |
The biological effectiveness of dosimetry-guided 131I therapy is not proven yet |
“Stunning effect” due to diagnostic activity of 131I could alter lesion biokinetics and the absorbed dose in a subsequent 131I therapy |
Conventional radiopharmaceuticals that allow quantitation of radioiodide uptake in thyroid remnants and metastases, such as 123I-iodide or 131I-iodide, have limitations; in fact, there are problems regarding cost and the logistics of supply for 123I-iodide, while 131I-iodide has poor imaging properties (that translate into relatively low sensitivity) and its use can cause the so-called “stunning” phenomenon [27]. In contrast, positron emission tomography (PET) with 124I-iodide provides images with high spatial resolution that can be corrected for both scatter and attenuation and therefore allows to accurately quantify tissue radioactivity.
Using the PET data as input to a fully three-dimensional dose planning program, Sgouros et al. [37] calculated spatial distributions of absorbed doses, isodose contours, dose-volume histograms, and mean absorbed dose estimates for a total of 56 tumors. The mean tumor absorbed dose for each patient ranged widely, from 1.2 to 540 Gy, while distribution of values for the absorbed dose in individual tumor voxels was even more dispersed, ranging from 0.3 to 4,000 Gy.
Median per patient tumor radiation absorbed doses between 1.3 and 368 Gy were reported by de Keizer et al. [38], who performed tumor dosimetry after rhTSH stimulated 131I-iodide treatment. Dosimetric calculations were performed using tumor radioiodide uptake measurements from posttreatment 131I scans, while tumor volumes were estimated from radiological images.
Besides being a promising method to obtain accurate dosimetry in patients with multiple metastases, 124I-iodide PET/CT is also a powerful diagnostic tool for accurate pre-131I-iodide therapy staging (superimposable to the post-therapy 131I-iodide WBS but without inducing the produce stunning effect) and for considering the usefulness of alternative therapies in high-risk patients.
Peptide Receptor Radionuclide Therapy
Peptide receptor radionuclide therapy (PRRT) represents a promising option for patients with somatostatin receptor expressing tumors, and several clinical trials have proven its efficacy especially for neuroendocrine tumors [39].
However, possible radiation-induced renal damage and large inter-patients’ variability in biodistribution and tumor uptake require accurate dosimetry-based therapy planning. Recent improvements in dosimetry methods and radiobiological models have resulted in promising results and correlations that constitute challenging perspectives for optimized PRRT and for other radionuclide therapies as well [39].
90Y-DOTATOC, 90Y-DOTATATE, and 177Lu-DOTATATE are the most widely employed radiopharmaceuticals for PRRT. Pilot trials also considered 111In-octreotide for therapy, in virtue of the high LET of its Auger-electron emission; however, since tumor response was seldom achieved, 111In-based PRRT protocols have virtually been abandoned [39].
The physical half-life of 90Y is compatible with the peptide kinetics, while the high energy of the β− particles confers high probability of killing all neoplastic cells in a certain volume around the uptake site through the cross-fire effect. However, 90Y is not suitable for imaging due to the lack of γ-emission, even if recent promising results have been shown for planar and SPECT Bremsstrahlung 90Y-images corrected for scatter and detector response, either based on Monte Carlo simulations or obtained by new generation equipments (SPECT/CT) [40, 41].
111In-octreotide, 86Y-octreotide, and 68Ga-peptides have been proposed as alternative options to imaging with 90Y. Due to their similar chemical characteristics, the assumption of comparable in vivo behavior of 111In- and 90Y-derivatives has been widely accepted [42]. Thus, several authors have used the identical molecule labeled with either 111In or 90Y for imaging or for therapy, respectively (e.g., 111In-DOTATOC and 90Y-DOTATOC). Since the physical T 1/2 of 111In is almost identical to that of 90Y and compatible with the distribution kinetics of peptides, the diagnostic activities usually administered (∼185 MBq) allow one to obtain serial images (planar, SPECT) over 3–4 days, although some difference in the chemical structure of 111In-octreotide makes it not perfectly adequate for dosimetry purposes [43].
The same compound labeled with 86Y (86Y-DOTATOC) is also a further option, since it totally preserves the chemical nature of 90Y derivatives and offers PET resolution. However, limitations of this approach are represented by the physical T 1/2 of 86Y (14.7 h, too short for adequately describing the kinetics of peptide uptake and retention), complex image quantification, cost, and poor availability [42].
Similarly, 68Ga-peptides are not suitable for dosimetry purposes, despite of the high-quality PET images provided, because of the extremely short T 1/2 of 68Ga (68 min) as compared to the kinetic pattern of distribution of peptides. Furthermore, the chemical properties of 68Ga might slightly alter the biodistribution kinetics of the radiolabeled peptide as compared to the therapeutic agent [42].
In comparison with 90Y, 177Lu induces less damage by cross-fire effect, but releases higher energy in smaller tissues. Furthermore, the γ-rays emitted by 177Lu are suitable for imaging and dosimetry. Nevertheless, for proper scintigraphic imaging, diagnostic/dosimetric activities of at least of 370–740 MBq should be administered. It should be noticed, however, that most often dosimetry is evaluated after administering therapeutic activities of 177Lu-DOTATATE (i.e., 3.7–7.4 GBq), as treatment is usually given in multiple cycles [39].
90Y- and 177Lu-peptides have shown similar biological half-lives for organs and lesions, with varying uptakes depending on the different expression of somatostatin receptors with respect to the peptide affinity. The same dosimetry methods and time schedule for data recording can be used in for 90Y- and 177Lu-PRRT. However, dosimetry must be assessed specifically for each radiopeptide used, and for the single patient, due to wide intra-patient variations as well as frequent inter-patient differences on tumor uptake. It should be noticed that the absorbed doses per unit activity for 177Lu-peptides are much lower (two- to fourfold) than for 90Y-peptides [44]. The activities administered in 177Lu- or 90Y-PRRT should vary accordingly, although the therapeutic protocols most commonly employed in clinical studies are not yet uniformly established with respect to activities administered and number of cycles [45].
Table 6.3 reports a summary of the dosimetric results for the most commonly used 90Y- and 177Lu-peptides (90Y-DOTATOC and 177Lu-DOTATATE), which share some basic aspects: (1) the pharmacokinetics shows very fast blood clearance and urinary elimination leading to low exposure of the whole body (Fig. 6.3), (2) the spleen, kidneys, and liver receive the highest absorbed doses, (3) the kidneys are the dose-limiting organs, (4) no significant uptake in bone or red marrow is typically reported.
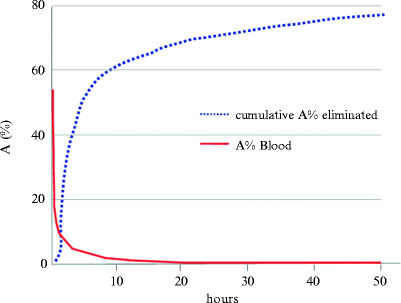
Table 6.3
Absorbed dose estimates for the principle PRRT trials with 90Y-DOTATOC and 177Lu-DOTATATE
Absorbed doses per unit activity (Gy/GBq) mean values among patients published by different authors | ||
---|---|---|
90Y-DOTATOC | 177Lu-DOTATATE | |
Red marrow | 0.03; 0.06; 0.09; 0.17 | 0.02; 0.04; 0.07 |
Kidneysa | 1.71; 2.44; 2.73; 3.84 | 0.62; 0.81; 0.88; 0.97 |
Liver | 0.27; 0.66; 0.75; 0.86; 0.92 | 0.13; 0.18; 0.21 |
Spleen | 2.19; 4.74; 5.36; 7.20 | 0.64; 1.2; 2.15 |
Bladder | 1.04; 1.59; 2.61 | 0.31 |
Total body | 0.10; 0.15; 0.28 | 0.05 |
Tumorsb | (1.4–31); (2.1–30); (2.4–42) | (0.6–56); (3.9–38) |
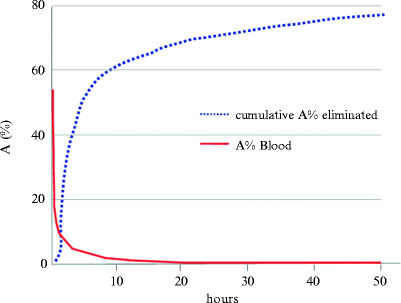
Fig. 6.3
Radiopeptides blood clearance (red curve) and urine excretion (blue dotted curve)
To reduce renal radiation burden preclinical and clinical studies showed that the infusion of protective agents (negatively charged amino acids) allows 25% to 65% reduction of the kidney uptake. Despite such protection, the cumulative dose to the kidneys could still be borderline with the limits considered for nephropathy induction, especially with 90Y-peptides. To improve accuracy of dosimetry estimates, the standard methods that assume uniform activity distribution in kidneys of standard masses have been shifted to the multi-region MIRD model for a sub-organ kidney dosimetry and dose-rate effects [46]. The analysis of PET or SPECT images can assign activity to the cortex or to the medulla in each single patient; more detailed information on intra-renal distribution can be also derived from the experimental results of renal autoradiograms [47]. The impact of the physical characteristics of the radionuclides with respect to inhomogeneous activity distribution (also within sub-organ regions) is a further issue under evaluation concerning potential nephrotoxicity.
A threshold biologically effective dose (BED) of 33 Gy for kidney radiation damage and a BED50 (defined as the BED value derived from the TD50 value derived from the EBRT) of 44 Gy were found for PRRT [46]. Besides the most relevant impact on PRRT, these findings have shown the great potential of sharing information from different radiation release modalities. As a further support for the radiobiological model, clinical experience has shown that multiple-cycle protocols lower the nephrotoxicity, while patients with high BED and more serious kidney side effects received the treatment in a few cycles [48]. This observation perfectly matches with the theoretical expectation from BED: for a given cumulative dose, the higher the number of cycles the lower the total BED, thus the lower the damage [42]. Clinical results have provided evidence of the safety up to a BED ∼40 Gy to the kidneys, cumulatively, and that risk factors (such as hypertension and diabetes) lower the tolerability to a BED ∼28 Gy [48].
Treatment protocols based on multiple therapy cycles represent a useful modality to lower toxicity, and/or improve the therapeutic outcome, owing to the different radiosensitivity of most tumors versus the kidney tissue. Cycles seemed especially effective in 90Y-PRRT, where frequent occurrence of renal impairment has been clinically confirmed [48, 49]. 177Lu-peptides and 111In-octreotide therapies appear to be less nephro-toxic [42, 48, 50]. Possible reasons for these different outcomes are the different particle range in tissues and different peptide localization. The short-range β-particles of 177Lu-peptides, or the Auger electrons of 111In-peptides, might irradiate more selectively the tubuli (radioresistant cells), while the long-range β-particles of 90Y-peptides may increase the irradiation of the glomeruli (radiosensitive cells), with consequent higher toxicity. Anyway, the issue of radionuclide toxicity is still to be elucidated [42]. Long-term data analysis of 177Lu-trials are not available yet, while a reliable comparison might require randomized trials conceived to release the same BED using different radiopeptides.
Individual differences in tumor mass and location, adjacent tissues, and targeting compound affinity could guide the choice of the most suitable radiopharmaceutical, also taking in mind that peptides internalize into the cells. In principle, the physical characteristics of 177Lu, with lower tissue penetration, makes it more suitable for small tumors and micro-metastases, while the cross-fire effect 90Y might have the advantage of a higher radiation burden to larger lesions [44, 45]. This hypothesis has been supported by preclinical findings and is laying the basis for new clinical study rationales proposing cocktails of 177Lu- and 90Y-radiopeptides.
Interestingly, a correlation has been observed between tumor absorbed dose and tumor response for 90Y-DOTATOC [51]. Responding tumors could be identified as those receiving much higher doses compared to nonresponding (up to sixfold: ∼230 vs. ∼40 Gy). Moreover, when correlating tumor absorbed dose versus lesion mass reduction, an encouraging trend for a dose–effect relationship was found, although not exactly a statistically significant correlation. Many parameters influence the response of tumors—such as tumor dimension, vascularization, radiosensitivity, and activity distribution—to make a strong dose–effect correlation easily ascertained. Larger series of data with specific radiobiological parameters for different tumor characteristics will certainly improve outcome prediction.
Radioimmunotherapy of Lymphoma
Radioimmunotherapy (RIT) offers an important option for the treatment of follicular low-grade non-Hodgkin’s lymphoma (NHL) refractory or relapsed after treatment with the current best practice [52]. Two radiolabeled antibodies are commercially available for treatment, tositumomab and ibritumomab, that are the radiolabeled immunoglobulin components of Bexxar and Zevalin, respectively [52, 53].
Bexxar is the 131I-labeled anti-CD20 antibody tositumomab, while Zevalin is the 90Y-labeled anti-CD20 antibody ibritumomab. Although both tositumomab and ibritumomab recognize the same epitope CD20 (one of many epitopes expressed on the mature B cell), they have slightly different binding characteristics [52].
For both radiopharmaceuticals, an unlabeled antibody is infused before administration of the radiolabeled murine anti-CD20 antibody component. In the Bexxar regimen, both the labeled and unlabeled antibody are tositumomab. In the Zevalin regimen, the unlabeled antibody is rituximab (the chimeric antibody used as an immunotherapeutic and marketed as Rituxan), while the labeled antibody is ibritumomab [52, 53].
In Europe only Zevalin is commercially available, while in the USA and Canada both Zevalin and Bexxar have been approved for clinical use [52].
No direct comparison in a randomized blinded trial has been performed to assess whether the difference in physical characteristics of the two radionuclides, 131I and 90Y, have different therapeutic effects or toxicity.
In principle, the greater energy of the β− emitted by 90Y is thought to be advantageous in the therapy of larger tumor masses, whereas the lower energy of the 131I particle emission is thought would be an advantage for treating patients with small tumor foci and marrow involvement. In the non-myeloablative setting, the use of either agent is contraindicated for patients with lymphomatous infiltration exceeding 25% of the bone marrow [54].
For Bexxar and Zevalin, different protocols and dose determinations have been reported, also due to the different approval requirements by regulatory agencies in the USA and Europe. However, for both radiopharmaceuticals, the red bone marrow is the dose-limiting organ due to its intrinsic radiosensitivity, to the rapid equilibration of the radiolabeled antibodies within its extracellular fluid volume, and to the long retention kinetics in the bone marrow [52].
When using Bexxar, dosimetry is estimated to determine the whole-body radiation-absorbed dose, since hematologic toxicity as well as the dose–clinical response relationship depend on the whole-body radiation-absorbed dose rather than on a MBq/kg dosing schedule [55].
The optimal clinical benefit with acceptable hematologic toxicity has been observed at 65–75 cGy whole-body radiation-absorbed dose, considering 65 cGy for patients with platelet counts between 100,000 and 150,000 and 75 cGy for patients with with platelet counts greater than 150,000 [52].
The whole-body radiation-absorbed dose is determined from three WBS acquisitions after the administration of 185 MBq of 131I-tositumomab (with prior infusion of 450 mg of unlabeled tostitumomab), administered for determining the residence time which is necessary for dosimetric estimates.
The purpose of the scan is simply to evaluate the whole-body total counts rather than to produce a diagnostic image, because the parameters involved in visualizing a tumor (dose administered, tumor size, and location) are different from those involved in radiobiological response evaluations [52].
Whole-body counts are determined from the total counts on the anterior and posterior WBS acquired 1 h postinjection (day 0), then 2 days (day 2), and 5 days (day 5) later, to determine the radiopharmaceutical residence time
and cumulated activity
in the body.


To calculate the desired therapeutic activity, the following equation can be used
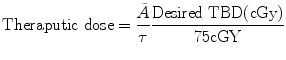
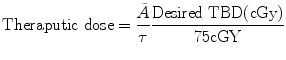
Seven to nine days after the initial “dosimetric” study, the infusion sequence (unlabeled tositumomab followed by 131I-tositumomab) is repeated with the 131I-tositumomab therapeutic activity (1.85–5.5 GBq) that had been determined by dosimetry.
Table 6.4
Organ radiation absorbed doses (mGy/MBq) for 90Y-ibritumomab tiuxetan (Zevalin®), based on pretherapeutic 111In imaging
Organ | Number of patients | Median and range (mGy/MBq) |
---|---|---|
Spleen | 166 | 7.35 (0.37–29.7) |
Liver | 179 | 4.32 (0.85–17.5) |
Lungs | 179 | 2.05 (0.59–4.86) |
Red marrow (blood derived) | 179 | 0.59 (0.09–1.84) |
Red marrow (sacrum derived) | 179 | 0.97 |
Kidneys | 179 | 0.22 (0–0.95) |
Bone surfaces | 179 | 0.53 (0.09–1.31) |
Urinary bladder wall | 179 | 0.89 (0.38–2.32) |
Other organs | 179 | 0.41 (0.06–0.62) |
Total body | 179 | 0.54 (0.27–0.78) |
In the US and EU countries, biodistribution studies are not required because of the lack of correlation between red marrow dose and toxicity, and considering that red marrow toxicity is reversible. This choice (which seems to be dictated mainly by practical/economical reasons) is arguable, as cases in which only the scintigraphic images were able to predict toxicity of the treatment are not so rare [58]. However, when using larger activities in clinical trials involving myeloablative doses with stem cell transplantation, personalized dosimetry estimates are required to avoid unpredictable toxicity. In fact, with bone marrow rescue it is not possible to identify a priori a “safe” maximal activity or a unique critical organ for all patients, because of the wide intra-patient variability in doses to the vital organs.
At present, neither 90Y-ibritumomab-tiuxetan dosimetry outcomes in favor of its predictivity have been found nor correlation between red marrow dose and hematologic toxicity has been demonstrated [55–57, 59, 60]. This consideration has discouraged the practice of red marrow dosimetry for Zevalin. In this regard, toxicity in these patients is most likely linked to involvement of bone marrow by the disease and to reduced bone marrow reserve due to previous aggressive chemotherapy regimens [61].
The therapeutic activity of Zevalin for the nonmyeloablative therapy of rituximab-refractory or relapsed patients with low-grade follicular non-Hodgkin’s lymphoma is prescribed proportionally to the patient’s weight, with recommended administered activity of 15 MBq/kg up to a maximum limit of 1,200 MBq [57]. Patients with platelet counts between 100,000 and 150,000 should receive 11 MBq/kg [57]. Much higher activities, ranging from 29 to 55 MBq/kg, have been used under myeloablative regimens, followed by autologus stem cell transplantation (ASCT) [62].
Radioembolization of Liver Tumors
Radioembolization (RE) is a locoregional treatment developed in order to release high radiation doses to malignant hepatic lesions by intra-hepatic administration of a radiation vehicle. The rationale is based on the fact that blood supply from the hepatic artery is almost excluded from the normal liver, while selectively provided to liver tumors. In the last decade, significant response rates have been achieved with this approach in patients with unresectable primary hepatocarcinoma (HCC) or secondary hepatic malignancies [63, 64].
Several RE techniques have been developed, including the administration of radiolabeled molecules (such as 90Y-,131I-,188Re-,166Ho-Lipiodol) or of microspheres loaded with radionuclides (e.g., 90Y-glass, 90Y-resin, 166Ho-polymer), differing in size, material, and specific radioactivity. The administration protocols depend on the selected radionuclides and on nature of the molecules or spheres. However, the amount of activity to be administered should generally be established taking into account the major factors potentially affecting the outcome of therapy. Among these are the tumor liver involvement, tumor uptake, possible side effects to healthy tissues, baseline patients’ condition, radiation dose delivery, and radiation protection impact. In practice, choice of the injected activity has been based on empirical approaches or on dosimetric evaluations, as summarized hereinafter for the most widely employed technique, which uses 90Y-microspheres.
There are two formulations of 90Y-microspheres: (1) TheraSphere (MDS Nordion, Ottawa, Canada) is composed of 15–30 μm nonbiodegradable glass microspheres with 90Y as an integral constituent of the glass [65]. It has been approved by the FDA for use in HCC patients with or without portal vein thrombosis. (2) SIR-Spheres (SIRTex Medical, Lane Cove, Australia) are composed of resin microspheres and have been approved for use in patients with colorectal carcinoma metastatic to the liver [66]. Both agents have regulatory approval for liver neoplasia in Europe and various countries worldwide.
For both, therapy is generally simulated by 99mTc-abumin macroaggregates (99mTc-MAA) administered intra-arterially, in order to establish any possible extra-hepatic shunting to the lungs or the gastrointestinal tract (GI) and to analyze the tumor (T) to non-tumoral liver (NTL) uptake ratio. Different approaches are used to establish the amount of activity to be injected for the two radiopharmaceuticals [64].
The biodistribution of 90Y-microspheres is usually predicted by 99mTc-MAA administration and subsequent imaging by planar and SPECT technique. The images are analyzed to estimate any possible shunting as well as to evaluate the absorbed doses to target and nontarget tissues. The adequacy of 99mTc-MAA for simulation is widely accepted, although not definitely proven. Although similar distribution patterns of 99mTc-MAA and Bremsstrahlung 90Y-microsphere images have been reported, some dissimilarities have also been observed, possibly due to the different nature of MAA versus microspheres or to occasional alterations of the original vascular anatomy produced by angiography [64]. Nevertheless, the acquisition of both pre- and post-therapeutic images represents a suitable source of information for risk/benefit evaluation. Patient-specific dosimetry requires a detailed evaluation of the mass from CT images and of the relative T/NTL uptake from SPECT or planar scans after intra-arterial administration of 99mTc-MAA. Using the standard dosimetry approach, mean values of the absorbed doses per unit activity can easily be derived by the OLINDA/EXM software, considering the “S factors” values specific for tumors, liver, and any other organ (e.g., lungs), properly rescaled for individual masses. A more sophisticated evaluation can be made by the voxel dosimetry approach or by Monte Carlo modeling, that provide information on dose distribution and expected radiobiologic effects at the voxel level [67].
Three main methods are suggested by the manufacturer [66] to calculate the activity of resin spheres (SIR-Spheres®): the empirical method, a body surface area (BSA)-based method, and the partition method.
The empirical method does not personalize the treatment for different liver dimensions or tumor avidity, but it takes into account the tumor involvement and attempts to lower the radiation risks to the lungs and the NTL. The activities calculated by the empirical method (based on the tumor involvement and the lung shunt fraction, LSF) are summarized in Table 6.5. In the same table, the absorbed doses to the lungs and to the NTL are also reported, evaluated for standard-size organs by applying the corresponding dose conversion factors [22]. With these activities (range: 1.2 to 3.0 GBq) the doses received by the lungs and by the NTL are within 18 and 83 Gy, at most.
Table 6.5
Radioembolization of liver tumors by resin spheres (SIR-Spheres®)
Tumor involvement | A (GBq) | Maximum absorbed dose to the lungs (Gy) | Maximum absorbed dose to the NTL (Gy) | ||||||
---|---|---|---|---|---|---|---|---|---|
LSF | LSF | LSF | |||||||
0–10% | 10–15%a | 15–20%b | 0–10% | 10–15% | 15–20% | 0–10% | 10–15% | 15–20% | |
≤25% | 2.0 | 1.6–2.0 | 1.2–1.6 | 10 | 12 | 12 | 50–55 | 37–50 | 26–37 |
25–50% | 2.5 | 2.0–2.5 | 1.5–2.0 | 12 | 15 | 15 | 62–69 | 47–62 | 33–47 |
>50% | 3.0 | 2.4–3.0 | 1.8–2.4 | 15 | 18
![]() Stay updated, free articles. Join our Telegram channel![]() Full access? Get Clinical Tree![]() ![]() ![]() |