Rapid Cardiovascular Magnetic Resonance Imaging
Mark Doyle
MOTIVATION
In the context of cardiovascular (CV) applications, rapid magnetic resonance imaging (MRI) is required primarily for two purposes:
To image the CV system in times less than physiologic time scales
To increase scan efficiency and patient throughput
There are several physiologic time scales that present a challenge to MRI, as follows:
Respiratory motion
Breath-holding; requires patient co-operation
Respiratory monitoring and synchronization; time consuming
Cardiac motion
Cardiac triggering; requires good electrocardiogram (ECG) signal
Real-time imaging; currently suffers from low resolution and/or contrast
Blood motion
Myocardial perfusion; contrast has a 20-second transit time
Contrast angiography; contrast has a 20- to 40-second transit time
Currently, conventional imaging applied to maximize speed is capable of providing diagnostic information, often of high quality. For example, in the area of angiography, a bolus of gadolinium-based contrast agent is imaged while in the arterial phase, allowing generation of a three-dimensional (3D) angiogram of a wide region. The acquisition time for this sequence is in the order of 20 to 30 seconds. In the area of myocardial perfusion imaging, the change in myocardial contrast as a contrast agent passes through is dynamically visualized. This requires a time resolution of one to two heartbeats, and is achieved while imaging multiple slices. One of the standard examinations performed by MRI is functional evaluation of the left ventricle (LV). This is typically accomplished in a breath-hold time to obtain a temporal resolution within the cardiac cycle of approximately 50 milliseconds.
Given this background, it may be queried why there is a requirement for yet more rapid imaging, because clearly, MRI can be used to acquire CV images of diagnostic value. Consider each of the major application areas itemized in the preceding text:
For angiography, correct synchronization of the angiographic imaging sequence with the arterial phase of the contrast agent is crucial to obtain good image quality. To this end, an angiographic timing sequence is typically run before the full acquisition. In the timing run, a small bolus is injected to calculate the time for contrast to reach the arterial system. This knowledge is used to activate the 3D acquisition. When the 3D acquisition is timed well, arterial blood contrast is excellent. However, when the 3D acquisition is timed badly, arterial blood contrast is poor. When poor contrast is obtained, at best, this may require a delay of several minutes to allow clearing of the agent, or at worst, the vascular information may not be acquired during that scan session (see Fig. 32-1). Sometimes, contrast is low due to aberrant flow patterns, and in these cases there is no single “correct” imaging time. The availability of rapid imaging would allow continuous imaging, thereby reducing setup time and improving reliability with the availability of time-resolved angiographic data.
For myocardial perfusion imaging, additional time is required in the cycle to generate contrast. Optimal contrast is not always generated due to acquisition time constraints, which often place an upper limit on the inversion time for the contrast pulse. Rapid imaging would allow improved contrast to be realized.
Although cine imaging of the LV can be accomplished in a breath-hold, the patient’s idea of a “comfortable
breath-hold” may be dramatically abbreviated from the “ideal” required for imaging. Therefore, rapid imaging can at the least reduce the breath-hold time, or at best eliminate the need for breath-holding if adequate quality could be obtained with a real-time approach.
![]() FIGURE 32-1 Example of adequate (left) and inadequate (right) vascular contrast for a 3D angiographic study using a bolus injection of contrast agent. The scan time and acquisition parameters for each scan were identical, the major difference being the timing of each scan relative to the passage of the contrast agent. This illustrates the sensitivity of the angiographic approach to timing. |
RAPID IMAGING APPROACHES
High-resolution images are usually preferable for diagnostic purposes. However, lower resolution images may yield “sufficient” information, the advantage being that lower resolution images can be obtained in less time. Further, it is possible to reduce the scan matrix while maintaining the resolution by the use of partial number of excitations (NEX). However, this approach reduces the signal-to-noise ratio (SNR). Alternatively, a partial phase field of view or rectangular matrix can result in fold-over artifacts. When using the multielement phased array coils it may be possible to deactivate one or more of the elements. This expediency can be used to reduce the effects of fold-over artifact, by removing the signal source. Special placement of active element(s) of the coil may be required for optimal imaging.
ECHO PLANAR IMAGING
Echo planar imaging (EPI) is a rapid gradient echo technique that employs a train of echoes, each one relating to a line of k-space. EPI can produce an image in approximately 50 milliseconds. EPI requires high performance gradients due to the multiple gradient switches required. However, because of the inherently long read out time for EPI, short T2 components tend to be blurred. A single-shot EPI image is generally of relatively low resolution, with matrices typically ranging from 642 to 1282. The major application of single-shot EPI to date has been in functional brain imaging, where the high T2 values, leading to strong T2 weighting, are an advantage. Currently however, application to cardiac use is limited.
MULTISHOT ECHO PLANAR IMAGING AND MULTIPLE ECHO GRADIENT ECHO
While gradient echo requires multiple applications of the basic imaging sequence to compile multiple lines of k-space, single-shot EPI generally has too long an echo train for cardiac applications. Therefore, a hybrid of the two has evolved, which acquires several lines of k-space for each basic imaging sequence. Echo train length (ETL) imaging utilizes a short train of echo signals, typically with values of 4 to 8. A series of four echoes (i.e., four lines of k-space) can be acquired with a repetition time (TR) of approximately 10 milliseconds. This is approximately a factor of 2 faster than the traditional gradient-recalled echo approach. The ETL approach can be combined with segmented scanning for CV imaging. Segmented ETL imaging is often used
to perform the rapid scanning required for myocardial perfusion imaging.
to perform the rapid scanning required for myocardial perfusion imaging.
SPIRAL SCANNING
A spiral trajectory in k-space can be accomplished by use of gradually increasing sinusoidal and cosinusoidal gradients (see Fig. 32-2). In principle a spiral scan could be obtained in a single shot, but more commonly, multiple interleaved shots are used. In the interleaved version, each spiral arm is approximately 20 milliseconds long, which is quite long for T2 blurring considerations. The spiral data requires regridding to accommodate the regular k-space matrix, which makes reconstruction times longer.
PARALLEL IMAGING APPROACH
The parallel imaging approach to reduce scan time relies on the use of a number of receivers positioned around the region of interest. Time reduction factors of 2 are common. Although a reduced field of view is used, the advantage to this approach is that no signal aliasing occurs (when properly applied).
K-SPACE SYMMETRY
K-space has the property of complex conjugate symmetry through the center (see Fig. 32-3). Using this property of symmetry, reduced scan times can be accomplished by acquiring reduced portions of k-space and filling in the portion not acquired by knowledge of the symmetry. Such an approach is exploited when a partial NEX value is used.
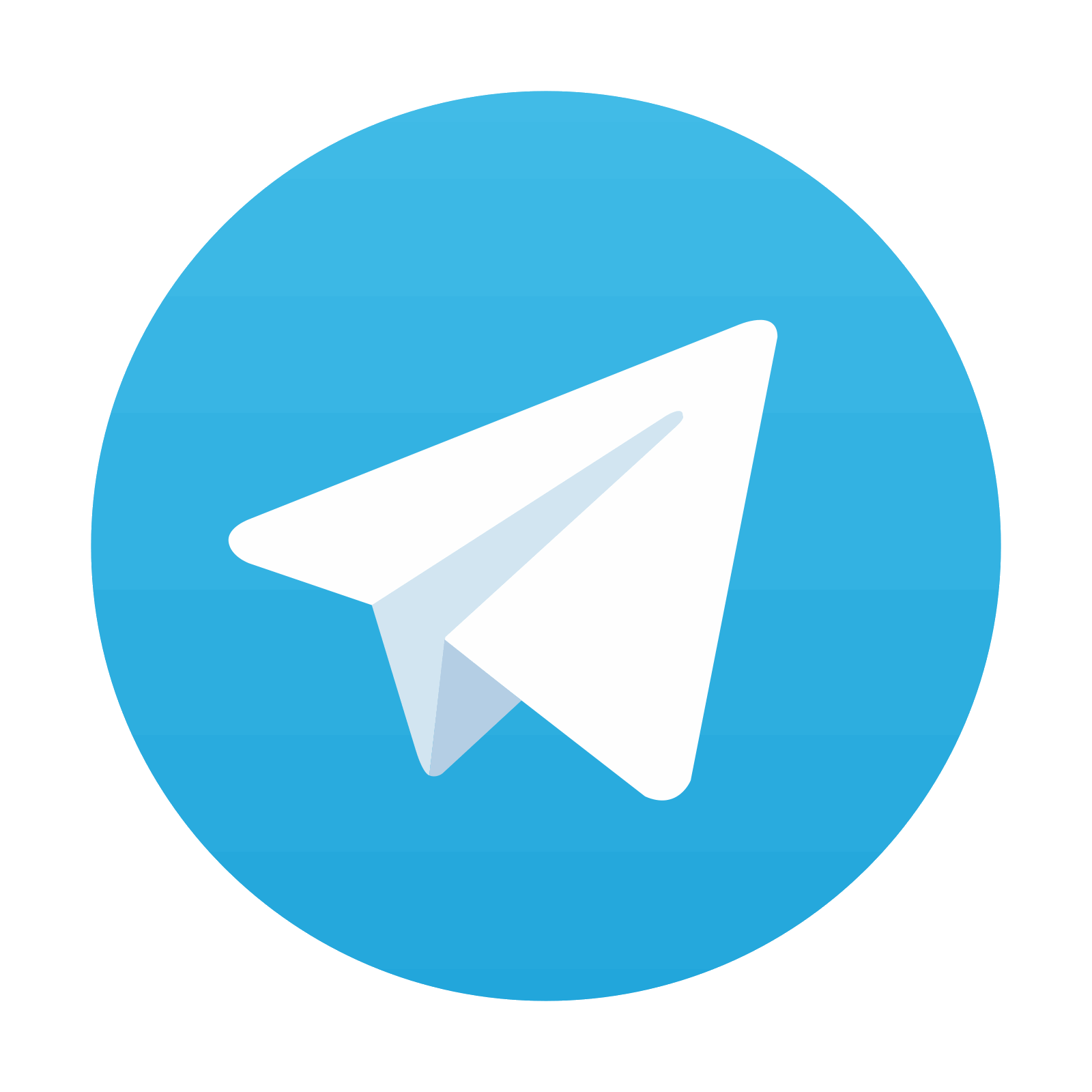
Stay updated, free articles. Join our Telegram channel
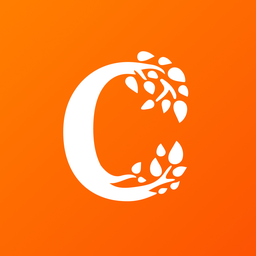
Full access? Get Clinical Tree
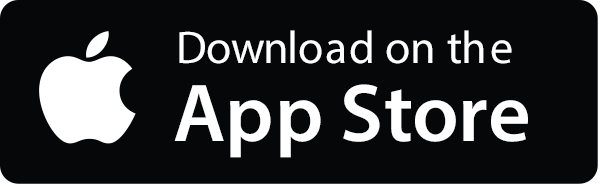
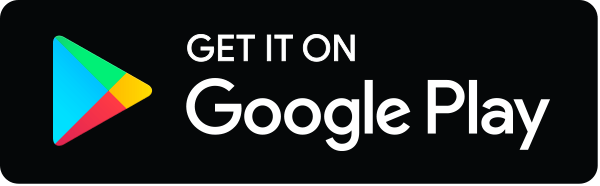