, M. Gaed2, 3, J. A. Gómez4, M. Moussa4, C. Romagnoli5, S. Pautler3, 6, 7, J. L. Chin6, 7, C. Crukley2, 3, G. S. Bauman3, 7, A. Fenster1, 2, 3, 7, 8 and A. D. Ward1, 3, 7, 8
(1)
Biomedical Engineering Graduate Program, The University of Western Ontario, London, ON, Canada
(2)
Robarts Research Institute, The University of Western Ontario, London, ON, Canada
(3)
Lawson Health Research Institute, London, ON, Canada
(4)
Department of Pathology, The University of Western Ontario, London, ON, Canada
(5)
Department of Medical Imaging, The University of Western Ontario, London, ON, Canada
(6)
Department of Surgery, The University of Western Ontario, London, ON, Canada
(7)
Department of Oncology, The University of Western Ontario, London, ON, Canada
(8)
Department of Medical Biophysics, The University of Western Ontario, London, ON, Canada
Abstract
Determining the intra-prostatic spatial distribution and grade of prostate cancer before treatment may support improved diagnosis, therapy selection, or guidance of intra-prostatic lesion-focused therapies (e.g., radiation boosting or ablative focal therapy). Several in vivo imaging modalities are showing promise for imaging the intra-prostatic distribution of cancer. Evaluations of such imaging modalities ideally include comparisons to registered histological examinations of prostatectomy specimens, the clinically accepted “gold standard” for staging and grading prostate cancer. The registration of histology to ex vivo magnetic resonance (MR) images supports these challenging in vivo registrations by reconstructing 3D spatial information that is lost during the process of acquiring histology sections. In the work described in this chapter, ex vivo MR and histology images were acquired from nine formalin-fixed radical prostatectomy specimens which had been marked with extrinsic fiducials designed to be visible in these modalities. The histology images were registered retrospectively to the MR images using a novel algorithm based on the minimization of fiducial registration error between fiducial cross-sections on histology images and parametric fiducial curves on MR images. The 3D target registration error (TRE) was quantified based on the post-registration misalignment of manually identified homologous landmarks (3–7 per section, 184 in total), and was compared to two previously developed methods: (1) a method based on the guidance of the coarse slicing of specimens and (2) a method based on additional imaging of the images of the thick tissue slices. The proposed method yielded a mean ± standard deviation TRE of 0.71 ± 0.38 mm, 0.38–0.63 mm lower [95 % confidence interval (CI)] than the image-guided-slicing-based method, and within 0.13 mm (95 % CI) of the tissue-slice-imaging-based method. One component of the proposed method was able to refine the result from the image-guided-slicing-based method to within 0.13 mm (95 % CI) of the proposed method. The proposed method also resulted in a 70 % decrease in specimen processing time compared to the image-guided-slicing-based approach previously implemented at our center.
Introduction
The noninvasive determination of spatial distribution and grade of prostate cancer before treatment has the potential to support improved diagnosis, therapy selection, and focal therapy. For example, the accurate assessment of tumor grade and extent is required to support the decision to pursue active surveillance versus radical therapy. The current standard of systematic biopsies may fail to sample areas of cancer and/or the areas of highest cancer grade. Biopsies accurately targeted to the most suspicious lesions may increase the accuracy of diagnosis based on histological examination of biopsy tissue. Beyond biopsy, application of conformal treatments to intra-prostatic lesion (gross tumor) volumes with focused therapy (e.g., radiation boost or ablative focal therapy) requires precise tumor delineation before therapy. Monitoring of response to treatment or progression of cancer for men on active surveillance currently relies on prostate-specific antigen testing, an indirect assessment of tumor response. Noninvasive monitoring of intra-prostatic disease could augment decisions regarding treatment for these men. All of these clinical applications could be supported by imaging.
Many imaging modalities are showing promise for staging or grading prostate cancer in vivo, including single positron emission computed tomography using prostate-specific membrane antigen (PSMA) antibody-based tracers (Prostascint) [1, 2], positron emission tomography using choline-based tracers (e.g., 11C-choline [3, 4] and 18F-fluorocholine [5, 6]) and multi-parametric magnetic resonance (MR) imaging, comprising T2-weighted [7, 8], dynamic contrast enhanced [9, 10], diffusion-weighted [11, 12], and spectroscopic [13, 14] MR protocols. The evaluation of these imaging modalities ideally includes a comparison to tissue histopathology from prostatectomy specimens, the currently accepted clinical “gold standard.” In the case of imaging of cancer distribution in the prostate, accurate registration of histopathology obtained from prostatectomy specimens with the in vivo imaging is required to assess the accuracy of the imaging in localizing and grading of intra-prostatic cancer foci.
Such imaging to histopathology registrations are challenging, in part, due to the processes involved in cutting histology sections from specimens for examination as part of current clinical protocols. For example, after radical prostatectomy, the prostate specimens are chemically fixed in formalin, causing tissue deformation and shrinkage. The fixed specimens are cut into 3–5-mm-thick slices, such that much of the spatial relationship between these pieces of tissue is lost. The slices are chemically treated to replace all water in the tissue with paraffin and are embedded in paraffin blocks, inducing further shrinkage. The paraffin blocks are mounted into a microtome cutting machine by hand, and oriented to align the front face of the tissue slice, seen through the translucent paraffin, with the microtome blade, introducing operator variability. Paraffin and tissue are sliced off the block until a full cross-section of tissue is visible (leaving as much tissue as possible in the paraffin block for medical records), and then a 4-μm-thick histology section is cut, resulting in a loss of 3D context and variability in the position and orientation within the tissue slice from which the histology section is taken. This section is unwrinkled and allowed to expand on a water bath before being mounted to a glass slide, potentially introducing further deformation. The deformation, loss of spatial information, and variability due to operators or processes, all present challenges to the registration of histology to in vivo images.
The techniques used to address these challenges fall into two major categories. Prospective techniques alter the processes involved in cutting histology sections from specimens to mitigate deformation, avoid loss of spatial information, or reduce variability. Retrospective techniques use assumptions about these processes and information (e.g., images or measurements) collected before, during, or after these processes to virtually undo the deformation, reconstruct lost spatial information, or account for variability. Many reconstruction methods use a combination of techniques from these two categories.
One common approach to the reconstruction of clinical specimens is to prospectively control the slicing of specimens into tissue slices [15–18], often using image guidance and specialized cutting devices to orient and position the cuts. These methods typically assume that histology sections are parallel and evenly spaced at a measured or pre-specified interval, an assumption usually supported by controlling the cutting of the initial tissue slices but undermined by the variability of the cutting process on the microtome [19]. An advantage of these approaches is that the position and orientation of the resulting histology can be pre-specified (to within the error of the image guidance and variability in the tissue slice and histology sectioning processes), allowing visual comparison of histological information from a single histology section with axis-aligned, rather than oblique, images. However, pre-specified tissue slice positions and orientations may not match those typically used in the standard pathology workflow and clinical reporting.
A second approach uses extrinsic fiducials and/or additional imaging to retrospectively reconstruct 3D spatial relationships of tissue sections lost during the histology acquisition, rather than prospectively constraining the spatial relationships. Extrinsic fiducials have been used to reorient histology images relative to one another [20, 21]. Additional imaging (typically photographs of tissue slices) has been used to account for tissue deformation [16, 22–25]. As in the guided-slicing approaches, these methods typically assume that histology sections are parallel and evenly spaced at a known interval, supported by prospectively controlling the cutting of tissue slices or retrospectively measuring them.
A third approach retrospectively reconstructs the spatial relationships between histology sections by registering histology images to 3D in vivo or ex vivo images of the intact prostate. Approaches that register directly to in vivo images hold the potential to reconstruct histology images with little disruption to the pathology workflow; however, they rely on the presence of intrinsic image information that may be disrupted by anatomic variability or even the disease processes of interest. Approaches that register to ex vivo images introduce some disruption to the pathology workflow due to additional imaging and have a similar reliance on intrinsic image information, but typically have more control over the imaging, allowing for images with a higher resolution and potentially more information in common with histology. Augmenting the intrinsic image information with extrinsic fiducials avoids the reliance on common image information (except for validation purposes), at the cost of additional disruption to the workflow to add the fiducials.
In the selection of appropriate methods for histology reconstruction, consideration of reconstruction accuracy is important. In the context of studies evaluating imaging modalities based on registration to 3D reconstructed histological information, reconstruction accuracy affects the studies’ statistical power [26, 27] (i.e., the likelihood of the studies finding true statistically significant effects). Because of this effect, the use of a method with higher reconstruction error in a given study creates a requirement for a larger sample size, which can increase the effort needed for patient accrual, expose additional subjects to nonstandard-of-care tests and interventions and, given the high per-patient cost of imaging evaluation studies, substantially increase the cost of a study (see section “Comparison with Other Algorithms” in the discussion for an illustration). Thus, it is important to consider the comparison of registration accuracy amongst potential reconstruction methods for such studies.
Our institution has previously developed two approaches for histology registration. We first developed a prospective guided-slicing-based method [17] that used extrinsic strand-shaped fiducials internal to the prostate, ex vivo MR imaging, and time-consuming image guidance of a magnetically tracked stylus to mark the desired cutting plane with the goal of obtaining histology images along pre-specified planes corresponding to the in vivo imaging planes. To eliminate the need for magnetic tracking and image-guided slicing, we developed a second retrospective method [28] that used both internal and external fiducials, as well as additional ex vivo MR imaging of the tissue slices, yielding accurate registrations that accounted for the variability of microtome cutting and allowed the pathologist to slice specimens according to the standard pathology workflow.
The goal of the work described in this chapter was to evaluate a novel retrospective registration based on the optimization of correspondence errors of internal and external fiducials between histology and ex vivo imaging that did not use additional imaging of tissue slices (simplifying the process and reducing cost and processing time). We compared the accuracy of this method to our previously developed methods. In particular, the intent of the comparison to the image-guided-slicing-based method was to elucidate whether the image-guidance procedures were necessary for accurate registration, and the intent of the comparison to the tissue-slice-imaging-based approach was to elucidate whether the additional imaging contributed to increased registration accuracy. Because the use of internal fiducial markers may be prohibited in some centers, representing a barrier to translation, we also compared the proposed method to a variation that omitted internal fiducials. The intent of this comparison was to measure the loss of registration accuracy resulting from the elimination of internal fiducials from the registration workflow. Finally, in order to facilitate the acquisition of histology sections corresponding to pre-specified imaging planes (necessary to display corresponding histological information from an entire histology section alongside an imaging-axis-aligned in vivo image, and not possible with the proposed algorithm alone), we evaluated whether refining the results from our image-guided-slicing-based method using the optimization of fiducial correspondence errors in the proposed method would improve the accuracy of the image-guided-slicing-based method. This combination could potentially yield the accuracy of the registration-based method and the control of image-guided slicing. For these comparisons, we quantified the target registration error (TRE) of the registrations using homologous intrinsic landmarks identified on histology and MR images. These evaluations can be summarized by five questions answered by this work, enumerated as follows.
1.
How accurate was the proposed method?
2.
How did the accuracy of the proposed method compare to that of our previous image-guided-slicing-based method?
3.
How did the accuracy of the proposed method compare to that of our previous tissue-slice-imaging-based method?
4.
How did the use of internal fiducials impact registration accuracy?
5.
Does applying fiducial correspondence error optimization to the image-guided-slicing-based method improve its accuracy?
This study was originally published in the Journal of Magnetic Resonance Imaging [29].
Materials and Imaging
As part of an ongoing prospective prostate imaging evaluation study, we obtained radical prostatectomy specimens from nine subjects. The inclusion criteria included: (1) aged 18 or older, (2) clinical stage T1 or T2 prostate cancer histologically confirmed on biopsy and (3) suitable for and consenting to radical prostatectomy. The exclusion criteria included: (1) prior therapy for prostate cancer, (2) use of 5-alpha reductase inhibitors within 6 months of the study start, (3) inability to comply with preoperative imaging, (4) allergy to contrast agents, (5) sickle cell or other anemias, (6) sources of artifact within the pelvis such as hip or penile prostheses, and (7) contraindications to MRI such as electronic implants, metal in the orbit or aneurysm clips. This study protocol was approved by the Human Subjects Research Ethics Board of our institution, and informed consent was given by all subjects.
Tissue Processing
An overview of the tissue processing for the study specimens is illustrated in Fig. 1. Prostate specimens were fixed in 10 % buffered formalin for 48 h immediately following surgery. Following fixation, extrinsic fiducials (described in detail in section “Fiducial Marking”) were applied to the specimen, and the marked specimen was imaged following the protocol described in section “Whole Specimen Ex Vivo MR Imaging.” As in our hospital’s standard prostate slicing protocol, the prostatic apex was removed and sliced parasagittally, the mid-gland was sliced into thick transverse slices, and the base was sliced parasagittally. Unlike our hospital’s standard protocol, the orientation of the cut removing the apex was guided to coincide with in vivo imaging for another study [17], the mid-gland was embedded in agar prior to slicing, the mid-gland was sliced on a rotary cutter to yield parallel 4.4 ± 0.2-mm-thick slices, and in preparation for whole-mounting, the slices were not quartered. Only the mid-gland tissue slices were used for this study. The tissue slices were imaged following the protocol described in section “Tissue Slice MR Imaging.” The tissue slices were decalcified and paraffin-processed following our hospital’s large specimen processing schedule. Four-micrometer-thick whole-mount histology sections were cut from the resulting paraffin blocks and stained with hematoxylin and eosin (H&E). The resulting histology slides were digitized following the protocol described in section “Digital Histology Imaging.”
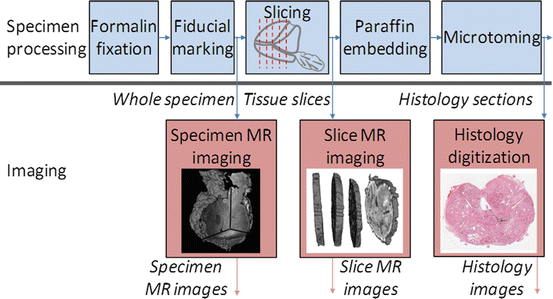
Fig. 1
An overview of the processing of the prostate specimens for histology and the imaging used in this study
Whole Specimen Ex Vivo MR Imaging
MR images of the intact prostate specimen (referred to as specimen MR images throughout this chapter) were acquired using a Discovery MR750 (GE Healthcare, Waukesha, WI, USA) at 3T using an endorectal coil (Prostate eCoil, Medrad, Inc., Warrendale, PA, USA). Specimens were positioned in approximately anatomical orientation in a sealed syringe, immersed in a fluorinated lubricant (Christo-Lube, Lubrication Technology Inc., Franklin Furnace, OH, USA) to reduce the proton signal surrounding the specimen and to eliminate artifacts. Specimens were imaged using two protocols: (1) a T1-weighted protocol used in the registration and accuracy evaluation that was sensitive to a gadolinium-based contrast agent in the extrinsic fiducials (3D SPGR, TR 6.5 ms, TE 2.5 ms, bandwidth ±31.25 kHz, eight averages, field of view (FOV) 140 × 140 × 62 mm, slice thickness 0.4 mm, slice spacing 0.2 mm, 256 × 192 matrix, 312 slices, flip angle 15°, 25 min) and (2) a T2-weighted protocol used only in the accuracy evaluation that was sensitive to anatomical detail (3D FSE, TR 2,000 ms, TE 151.5 ms, bandwidth ±125 kHz, three averages, FOV 140 × 140 × 62 mm, slice thickness 0.4 mm, slice spacing 0.2 mm, 320 × 192 matrix, 312 slices, 25 min).
Tissue Slice MR Imaging
MR images of the 4.4-mm-thick tissue slices (referred to as tissue slice MR images throughout this chapter) were also acquired. Tissue slices were immobilized with gauze in tissue-processing cassettes and immersed in Christo-Lube within a plastic bag. The tissue slices were imaged with the same MR scanner and coil as the specimen MR images, using the same MR protocols adjusted for a larger FOV to allow for spacing between slices and for the thickness of the cassettes.
Digital Histology Imaging
H&E-stained histology slides were digitized on a ScanScope GL (Aperio Technologies, Vista, CA, USA) bright field slide scanner at a 0.5 μm pixel size. These images were downsampled to yield images with a 0.03 mm pixel size (referred to as histology images throughout this chapter) for interactive fiducial and landmark localization (described in detail in sections “Fiducial Marking” and “Registration Accuracy”).
Methods
Fiducial Marking
Prostate specimens were marked with two previously reported [17] types of strand-shaped fiducial markers designed to be visible on specimen MR and histology images: (1) external fiducial markers affixed to the surface of the specimen and (2) internal fiducials passed through the specimen. The strand shape of the markers ensures the fiducials reliably intersect the thin histology planes. Fiducials were laid out as shown in Fig. 2. This layout was chosen such that the spatial configuration of fiducials intersecting with two different planes (i.e., two potential histology-specimen MR image alignments) could be distinguished under an affine transformation. Neighboring external fiducials, with the exception of fiducials 1 and 2 used to mark anterior left, were placed at approximately 45° angles relative to each other, so that the spatial configuration was sensitive to inferior–superior displacement and oblique orientation of the planes.
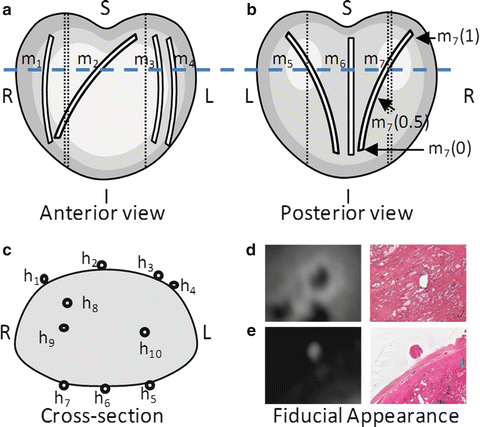
Fig. 2
An (a) anterior and (b) posterior view of a prostate showing the schematic layout of strand-shaped fiducials on the specimen, showing external fiducial curves m 1 through m 7, with internal fiducials m 8, m 9, and m 10 shown as unlabeled dotted lines. (c) Cross-sectional view corresponding to dashed line in panels (a) and (b), showing fiducial cross-sections h 1 through h 10. (d) Internal and (e) external fiducials appearance on T1-weighted specimen MR and histology images
The external fiducials comprised cylinders of cortex tissue from lamb kidney ~30 mm in length and 1 mm in diameter (extracted using the cannula of a biopsy needle), soaked in a 1:40 solution of gadolinium-based contrast agent (Magnevist, Bayer AG, Germany) to 10 % buffered formalin. These fiducials were affixed to the surface of the specimen with a toughened, heat-resistant, ethyl cyanoacrylate glue (Loctite 411, Henkel Inc., Germany). The use of tissue as the fiducial material enabled the fiducials to adhere to the positively charged glass when the histology sections were mounted to slides, as tissue is typically negatively charged. Kidney cortex tissue in particular had material properties that were conducive to its use as a fiducial: (1) it was firm enough to stay intact through the physical and chemical processes of fiducial application and histology processing, (2) it was soft enough to avoid scoring the histology section when cut on a microtome, and (3) its appearance on histology images was distinct from that of prostate tissue, supporting fiducial localization. The gadolinium-based contrast agent enabled the fiducial to be visible on T1-weighted specimen MR images. The appearance of the external fiducials on T1-weighted specimen MR and histology images is shown in Fig. 2e.
The internal fiducials comprised cotton threads soaked in a 1:40 solution of gadolinium-based contrast agent to blue pathologist’s ink (Tissue Marking Dye, Triangle Biomedical Sciences Inc., Durham, NC, USA). These threads were introduced through the specimen by passing a Quincke tip 18-gauge spinal cannula with stylet (BD Medical Inc., Franklin Lakes, NJ, USA) through the prostate, removing the stylet, passing the soaked cotton thread through the cannula, and removing the cannula, leaving the thread in place. The cotton thread was left in place during MR imaging and removed prior to tissue slicing. The gadolinium-based contrast agent and pathologist’s tissue ink supported the visibility of the internal fiducials on the MR and histology images, respectively. The Quincke tip cannula has a bevel to one side that results in tissue being separated and moved laterally instead of being damaged. The appearance of the internal fiducials on T1-weighted specimen MR and histology images is shown in Fig. 2d.
The external and internal fiducials were interactively localized on the T1-weighted specimen MR images and histology images. On histology images, centers of the cross-sections of external and internal fiducials were manually selected in 3D Slicer (Surgical Planning Lab, Harvard Medical School, Boston, USA). On specimen MR images, the endpoints of the fiducials were manually selected in 3D Slicer, and the centerline of the entire fiducial strand was automatically computed using a fast-marching algorithm [30]. For some histology images, a subset of the fiducials were not visible, either due to an external fiducial being lost during processing or due to the histology section being taken from a plane past the endpoint of the fiducial. These histology sections were reconstructed using only the remaining visible fiducials.
Histology Registration
Each histology image was registered by minimizing the fiducial registration error (FRE) between the fiducial cross-sections on the histology image and the fiducial centerline on the corresponding specimen MR image over the space of affine transformations. Unlike minimizing the FRE of two sets of points over the affine transformations, minimizing the FRE between a set of points and a set of curves cannot be solved analytically. As this minimization may have local minima, and an exhaustive search of the nine-dimensional affine transformation space is computationally expensive, the minimization was instead performed using a three-step optimization: (1) identifying a plane in the specimen MR image for initialization using a discretized exhaustive search in a low-dimensional space, (2) computing a point-wise correspondence based on the identified plane between fiducial cross-sections on histology images and points along the fiducial strand on specimen MR images, and (3) optimizing the FRE iteratively in a high dimensional space. While steps 1 and 2 are closely linked, separating these two steps allows a more direct comparison to existing registration methods that can yield a slicing plane as an output. This algorithm is described formally in the following sections.
Reconstruction Algorithm Notation
The center point of the cross-section of the i-th fiducial on a histology image is denoted by the 2D point h i (illustrated in Fig. 2c). The centerline of the i-th fiducial strand on a specimen MR image is denoted by the 3D parametric curve m i (s i ∈ [0,1]) (illustrated in Fig. 2a, b). Each point h
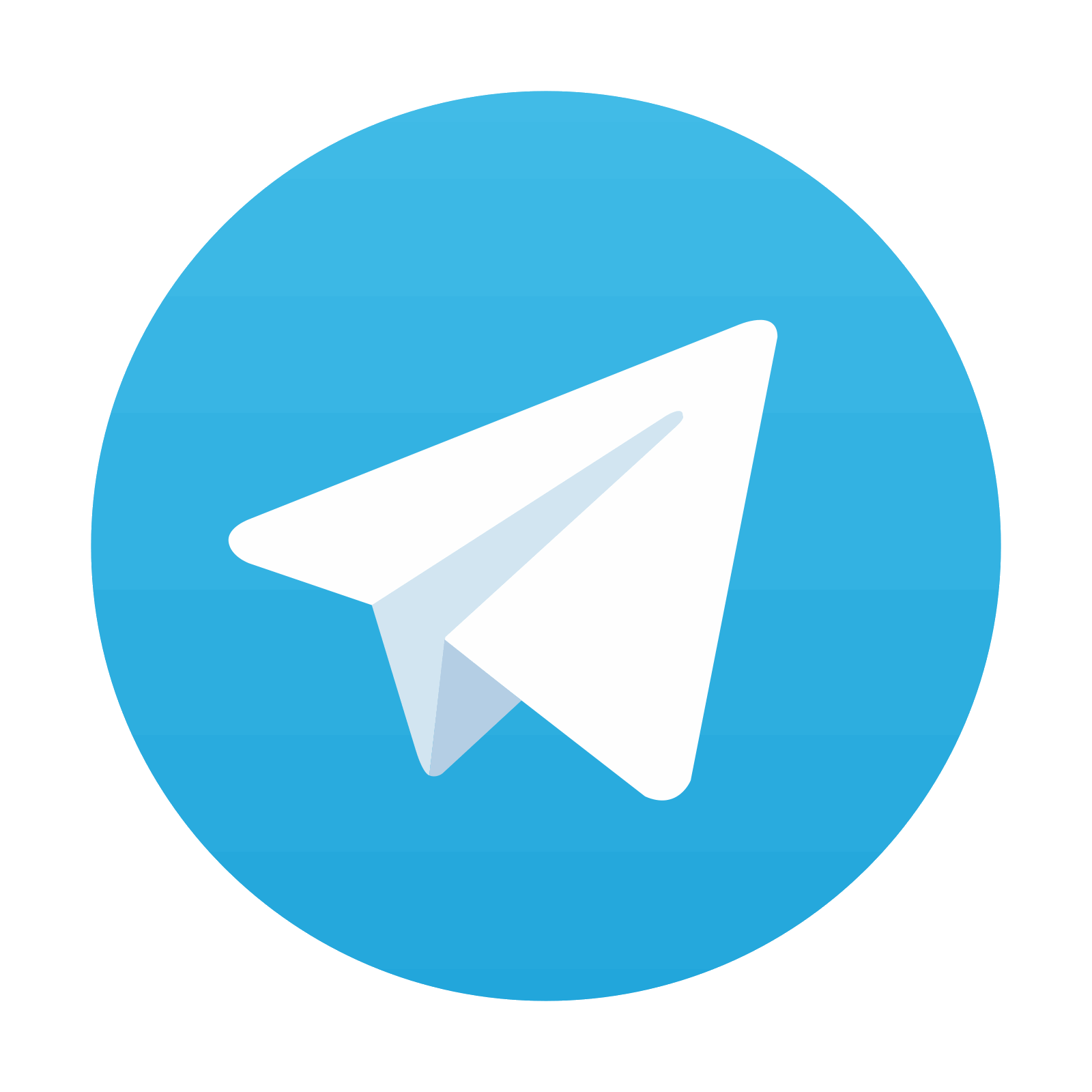
Stay updated, free articles. Join our Telegram channel
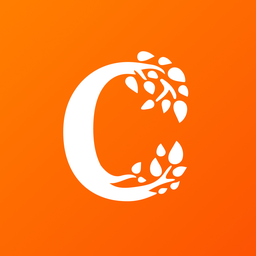
Full access? Get Clinical Tree
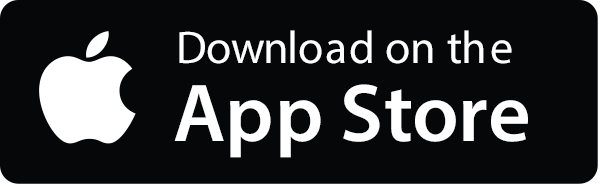
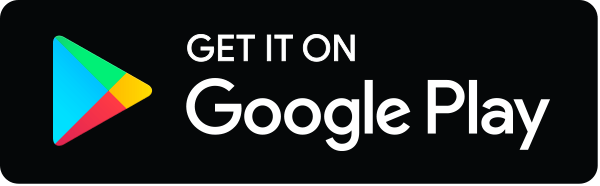