Fig. 3.1
Forty-four-year-old woman with stage IVA cervical cancer with pelvic lymph node metastasis. Sagittal (a) and axial (b, c) T2-weighted images demonstrate the tumor (arrow) invading the posterior bladder wall and an external iliac lymph node on the right (arrowhead). Fused diffusion-weighted and T2-weighted images (d, e) demonstrate restricted diffusion in the tumor (arrow) as well as in the metastatic lymph node (arrowhead)
To provide “multiparametric MRI,” MRSI and DW-MRI are at times used in conjunction with each other or with dynamic contrast-enhanced MRI (DCE-MRI) [8]. For DCE-MRI, images are acquired repeatedly as a contrast agent passes through tissue, providing potentially useful information about blood flow and vascularization. With certain cancer treatments, changes in tumor vascularity that reflect treatment response may be visible on DCE-MRI before tumor size changes can be discerned on anatomic imaging. Thus, DCE-MRI appears to be a promising source of predictive biomarkers and has been used for early treatment response assessment in patients treated with anti-angiogenesis drugs, chemotherapy, and other therapies [15–19]. The role of DCE-MRI in clinical practice has been limited by the relatively small number of patients in many published trials, the use of widely varying acquisition techniques and modeled parameters, as well as the use of diverse disease endpoints. Current attempts to standardize DCE-MRI will help to address these issues in the future [8, 20].
Novel technologies for in vivo molecular imaging include new tools for Raman spectroscopy, which can distinguish specific molecular compositions based on the wavelengths of photons with which they have interacted [21]. In addition, equipment for a process called “dynamic nuclear polarization” (DNP) has just entered the clinical research arena [22]. MR signal is proportional to nuclear spin polarization (the difference in the fraction of nuclei aligned with or against an applied magnetic field); such polarization is typically very small, making it a challenge to image nuclei other than protons, which are readily apparent only because of their natural abundance in the body [22]. DNP makes it possible to image nuclei such as 15N or 13C rapidly and with high sensitivity by temporarily “hyperpolarizing” them [22]. After the administration of a hyperpolarized agent such as [1-13C]pyruvate, the agent itself, as well as its metabolic products, can be depicted with MRI technology.
At present, PET (which is typically combined with CT to provide anatomical context) is the most versatile molecular imaging technology available. Not only can it detect and quantify abnormal molecular activity throughout the body, it can be applied with a wide range of molecular imaging probes [23].
3.2 Current and Emerging Applications for Molecular Imaging in Oncology
The most important imaging modalities in oncology today are CT, MRI, and PET/CT. Compared to CT and PET/CT, MRI has important advantages, such as the lack of radiation exposure, high spatial and temporal resolution, superior soft tissue contrast, and the capacity for multiparametric imaging. Though it is still limited to the research setting, MRI with hyperpolarized molecules allows detailed studies of tumor metabolism not only in preclinical models but also in humans. MR/PET imaging (Fig. 3.2) offers the potential for robust, joint structural, functional, and molecular imaging assessment of a wide variety of tumors, as it allows PET techniques to be combined with the broad array of MRI techniques – including hyperpolarized MRI. As such, it promises to markedly enhance cancer detection and characterization, treatment planning, response prediction, and response assessment, and it could help optimize the development of new drugs by noninvasively providing in vivo quantitative data about their pharmacokinetics and pharmacodynamics [24]. Where possible, the substitution of MR/PET for PET/CT would also reduce radiation exposure, which is highly desirable for all patients and especially for pediatric patients and those who undergo multiple examinations.
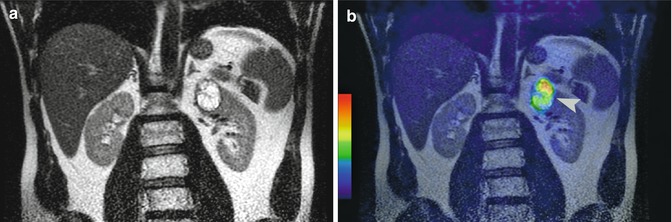
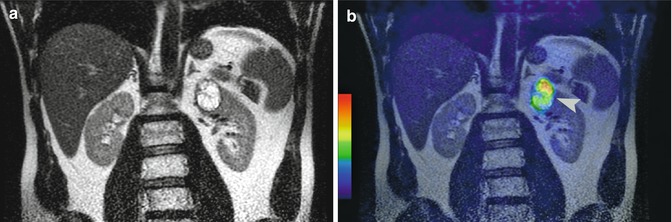
Fig. 3.2
Patient with clear cell carcinoma, Fuhrman grade II/IV. Coronal T2-weighted MR image (a) shows a renal mass at the left upper pole. Fused iodine-124-labeled cg250 PET/T2-weighted MR image (b) demonstrates tracer uptake within this renal mass (arrowhead). Pathology confirmed the presence of a clear cell carcinoma
3.2.1 Imaging in the Era of Genetic Medicine
Developing and validating quantitative imaging biomarkers that reflect underlying histologic and/or genomic features may provide a novel way of noninvasively assessing tumor heterogeneity and predicting drug response or resistance in oncology. The advent of molecularly targeted therapy and anti-angiogenic strategies has heightened the need for sensitive biomarkers for predicting treatment response and outcome, as resistance to chemotherapeutics and targeted therapies is common. Genetic intratumoral heterogeneity may contribute to treatment failure by initiating phenotypic diversity that introduces tumor sampling bias and enables drug resistance to emerge. In patients with primary and metastatic renal cell carcinoma, multiregion genetic analyses showed that spatially heterogeneous somatic mutations and chromosomal imbalances lead to phenotypic intratumoral diversity and that a single tumor biopsy specimen reveals a minority of the genetic aberrations present in an entire tumor [25]. Using phylogenic tree analysis to evaluate the relationships between tumor deposits in patients with ovarian cancer, Cowin et al. [26] found substantial copy number differences between metastatic deposits within individual patients and identified signaling pathways plausibly linked to peritoneal dissemination and establishment of metastatic foci. Significantly greater genomic change was observed in patients who experienced relapse after responding to chemotherapy than in patients who were resistant from the outset, possibly reflecting the requirement for selection of a subpopulation of resistant cells in cases initially sensitive to treatment [26].
Morphological heterogeneity between and within tumors is readily apparent in clinical imaging; subjective expressions of these differences, such as spiculated, enhancing, and necrotic, are common descriptors in the imaging lexicon. In the past several years, imaging research has focused on quantifying these image features in an effort to understand their biological and clinical implications. More recently, in an approach referred to as “radiogenomics,” quantitative shape, border, and texture analysis features (radiomics) obtained from CT, MRI, and PET have been correlated with genomic data and outcome data with the goal of developing robust biomarkers. Following extraction of more than 100 CT imaging features, Segal et al. [27] found that a subset of 14 features predicted 80 % of the gene expression pattern in hepatocellular carcinoma. A similar extraction of features from MRI of glioblastoma was able to predict protein expression patterns [28]. Radiomic features such as texture can predict response to therapy in renal cancer [29] and overall survival in primary [30] and metastatic colon cancer [31]. Analysis of heterogeneity on DCE-MRI and DW-MRI has been shown to improve diagnosis and assessment of prognosis for several tumor types [32–35]. Furthermore, measures of metabolic heterogeneity at baseline PET were able to predict response to chemoradiotherapy in patients with esophageal cancer [36].
Although radiomics analyses have shown a high degree of prognostic power, they are not spatially explicit. Radiomic features are generated over the entire tumor, using the assumption that tumors are heterogeneous but well mixed. However, it is readily apparent from DCE-MRI that perfusion can vary markedly within spatially distant tumor subregions that may represent distinct phenotypic habitats. Therefore, image-guided multiregional tumor analysis may be required to fully characterize tumor heterogeneity.
3.2.2 Contributions of Molecular Imaging in Treatment Selection and Planning
Optical imaging techniques have been developed to guide minimally invasive procedures (e.g., involving endoscopy or robotic techniques) as well as conventional surgical procedures. The use of fluorescence during routine endoscopic screening examinations in the gastrointestinal, bronchial, and urinary tracts is proving to be invaluable for detecting occult dysplastic lesions [37]. Furthermore, intraoperative optical imaging using fluorescence has been applied for tumor margin delineation and to identify malignant nodes. For example, optical imaging after the injection of indocyanine green (ICG) has been used to delineate tumors in patients with brain cancers as well as to map sentinel lymph nodes in patients with breast cancer [38, 39]. Recent research suggests that in ovarian cancer, tumor-specific intraoperative fluorescence imaging using a folate receptor-targeted fluorescent agent may improve intraoperative staging and allow more radical cytoreductive surgery [40].
Nanoparticle-based probes that contain more than one type of targeting component offer possibilities for applying multiple molecular imaging modalities in quick succession to improve gross tumor resection as well as subsequent fine margin resection. For instance, in studies using animal models, one probe tested allowed gliosarcomas to be identified by both preoperative MRI and intraoperative optical imaging [41], while another allowed visualization of glioma by MRI, photoacoustic imaging, and Raman imaging [42].
Molecular imaging can play an important role in radiotherapy planning. Higher radiation doses can be selectively applied in areas of tumor that show high metabolic activity and are therefore likely to represent especially aggressive disease. For example, FDG-PET/CT-based radiation planning for lymph nodes has been found to change the radiotherapy field in 21–25 % of patients with lung cancer [43–45]. In a study of 41 patients with head and neck cancer, the radiation boost dose was markedly increased and targeted at the tumors with the highest FDG avidity [46]. The study indicated that a boost dose of 3.0 Gy/fraction to ≤10 cm3 could be safely applied to the FDG-avid regions during the first 2 weeks of conventionally fractionated IMRT. A majority of local relapses occurred within the FDG-avid regions that had received elevated doses, suggesting that FDG avidity is a marker of the likelihood of relapse [46]. Randomized controlled trials comparing conventional and PET-based radiotherapy of locally advanced non-small cell lung cancer are ongoing (PETPLAN, NCT00697333; RTOG-1106, NCT01507428). These trials will investigate whether local control rates and side effects are improved by PET-based radiation treatment planning.
Fluoro-l-thymidine (FLT)-PET/CT can noninvasively measure tumor cell proliferation and can detect early radiotherapy response. For example, in head and neck cancer patients, rapidly decreasing FLT-PET SUVs have been observed during the course of radiotherapy. Conceivably, the radiation dose could be boosted in selected tumor areas with high proliferative activity identified by FLT-PET [47].
In organs such as the brain, where the accuracy of FDG-PET/CT is limited, MRS or PET with radiolabeled amino acids can provide an alternative means for guiding radiotherapy based on molecular information. The presence of metabolic abnormalities on MRS consistent with tumor outside of the target volume of Gamma knife radiosurgery is associated with significantly shorter overall survival [48]. Tumor volumes defined by PET with the radiolabeled amino acid analog fluoroethyltyrosine (FET) are markedly different from those defined by contrast enhancement or FLAIR signal abnormalities on MRI (Fig. 3.3) [49, 50]. A randomized controlled trial comparing amino-acid PET-based radiation treatment planning with MRI-based radiation treatment planning is ongoing (GLIAA, NCT01252459). This trial will determine whether progression-free survival is improved by PET-based radiotherapy.
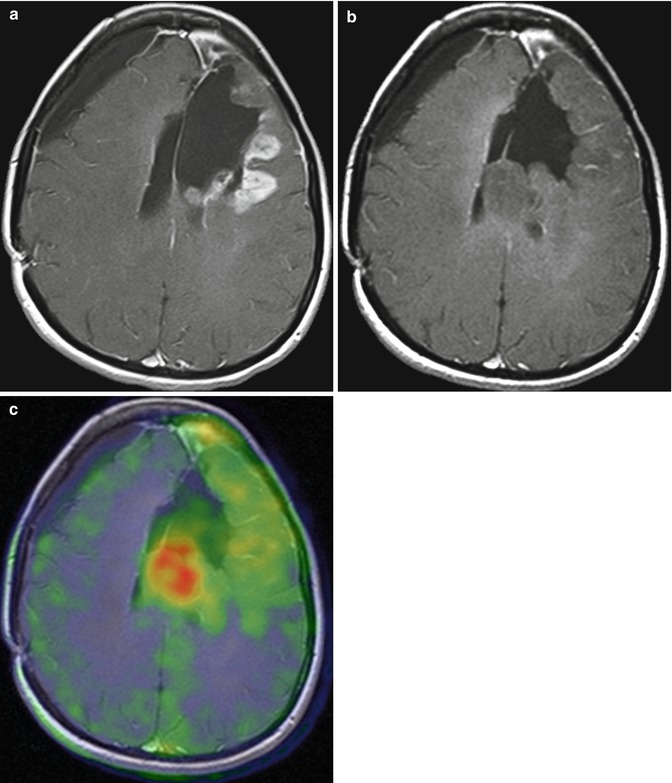
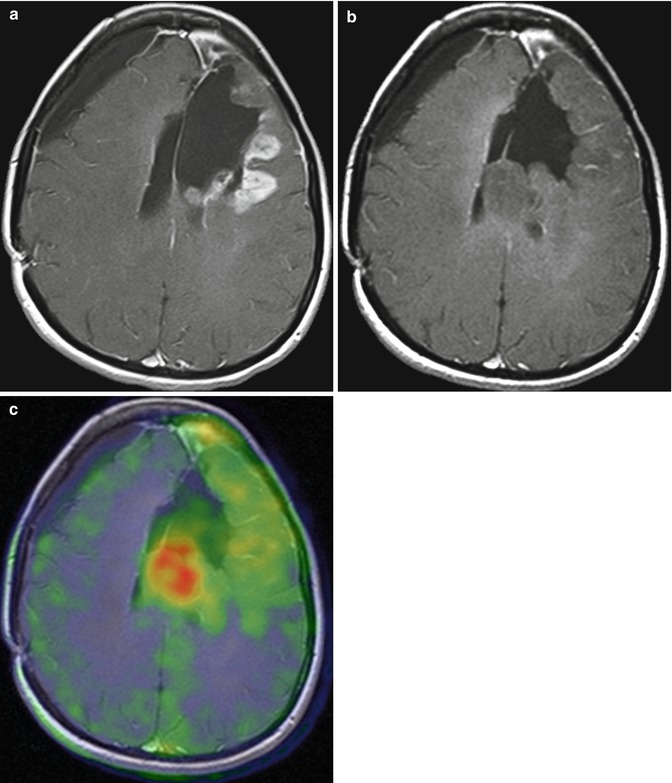
Fig. 3.3
Value of PET with 18F-fluoroethyltyrosine (18F-FET) in recurrent glioblastoma. T1-weighted MRI (a) shows a left frontal contrast-enhancing lesion, lateral to the resection defect. After 2 months of treatment with bevacizumab, T1-weighted MRI (b) shows a marked reduction of contrast enhancement but a growing tumor mass. 18F-FET PET demonstrates metabolically active, viable tumor tissue in the left frontal lobe as well as adjacent to the left ventricle (c), illustrating the ability of 18F-FET PET to depict the infiltrative growth of brain tumors
Various techniques are available for imaging tumor hypoxia, which increases resistance to radiotherapy and chemotherapy [51, 52]. These techniques include PET with tracers such as [18F]fluoro-misonidazole (FMISO), [18F]fluoroazomycin-arabinofuranoside, or [60/64Cu]copper(II)-diacetyl-bis(N4-methylthiosemicarbazone (ATSM), as well as blood oxygen level-dependent (BOLD) MR imaging, which detects hypoxia based on an increase in the transverse relaxation rate (R2*) of water caused by the paramagnetic effect of endogenous deoxyhemoglobin [53, 54]. Studies are ongoing that evaluate whether local control rates can be improved by applying higher radiation doses to hypoxic tumor subvolumes [55]. However, it remains an open question whether the size and location of hypoxic volumes remains stable in untreated tumors and during radiotherapy.
Imaging of hypoxia has also shown promise for guiding the use of chemotherapeutic drugs that specifically act on hypoxic tumors (Fig. 3.4): The hypoxia-selective drug tirapazamine was not beneficial in unselected head and neck cancer patients but only in the subgroup of patients with FMISO-PET-positive tumors [56, 57].
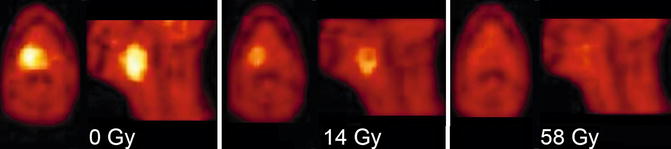
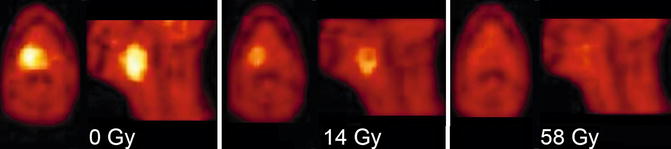
Fig. 3.4
Monitoring of tumor hypoxia in oropharyngeal cancer during radiotherapy with 18F-fluoro-misonidazole (18F-FMISO) PET (axial and sagittal PET images are shown). The untreated tumor (0 Gy, left) shows high tracer uptake indicating the presence of hypoxia. During treatment (14 Gy, middle, and 58 Gy right) there is a rapid decrease of FMISO uptake consistent with resolution of hypoxia
3.2.3 The Role of Molecular Imaging in Treatment Follow-Up
It is essential to be able to detect and localize residual and recurrent cancers so that timely and appropriate salvage therapy can be administered. However, posttreatment changes (e.g., loss of normal anatomy or the formation of scar tissue) often hamper the identification of such cancers by conventional cross-sectional imaging. By adding metabolic to morphologic information, molecular imaging can help to distinguish recurrent or residual disease. The use of FDG-PET for further evaluation of patients with clinically suspected tumor recurrence is well established in many malignancies including head and neck squamous cell carcinomas, lung cancer, rectal cancer, and ovarian cancer. In these patients PET/CT can guide local therapeutic strategies such as resection of solitary liver metastases [49, 58–63]. However, the role FDG-PET for surveillance in asymptomatic patients needs further study. In patients with a low risk of recurrence, the positive predictive value of FDG-PET may be too low to be clinically useful. Conversely, it has not been proven in randomized trials that early detection of asymptomatic recurrences by FDG-PET improves outcome.
MRS can also be a useful supplement to anatomic imaging in the search for recurrence. Studies have demonstrated its ability to help differentiate locally recurrent prostate cancer from benign and necrotic tissue after radiation treatment (Fig. 3.5) [65], hormone deprivation therapy [66], and cryosurgery [67]. DW-MRI and DCE-MRI are also very useful in evaluating local recurrence of prostate cancer and have been incorporated in routine clinical practice (Fig. 3.6) [68, 69].
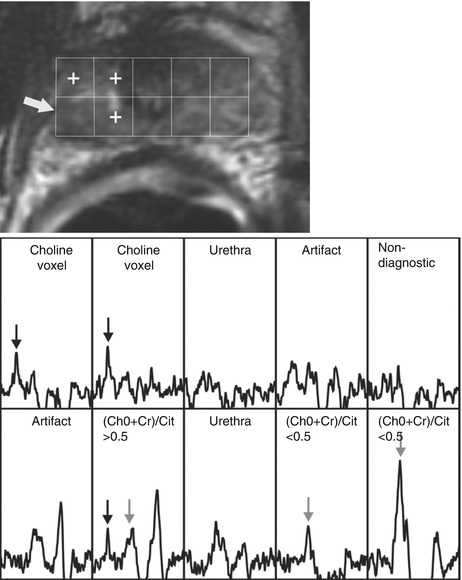
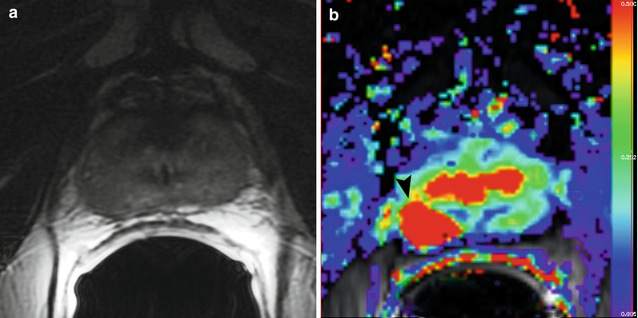
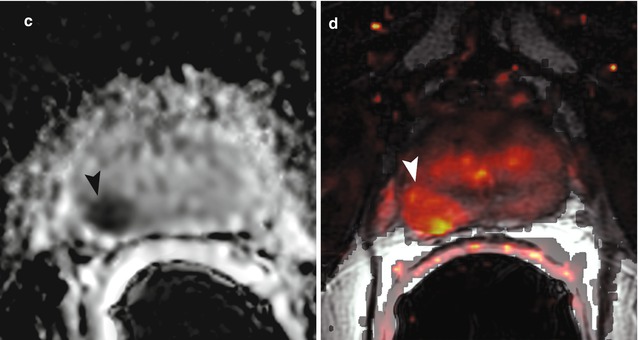
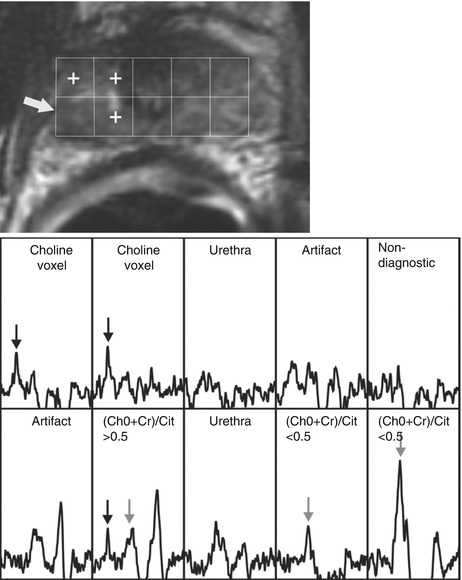
Fig. 3.5
Local prostate cancer recurrence after external beam radiation therapy, detected with MR imaging and MR spectroscopy. This 63-year-old patient had increasing PSA levels 66 months after completion of external beam radiation therapy. (a) Transverse T2-weighted MR image (4000/96, 14-mm field of view, 3.0-mm section thickness, no intersection gap, 256 × 192 matrix, and four signals acquired) with overlaid MR spectroscopic grid; spectra obtained in the prostate apex are shown in (b). A suspicious focal nodular region of reduced signal intensity at T2-weighted MR imaging was observed on the right side (arrow in a) (Adapted and reprinted from Pucar et al. [64])
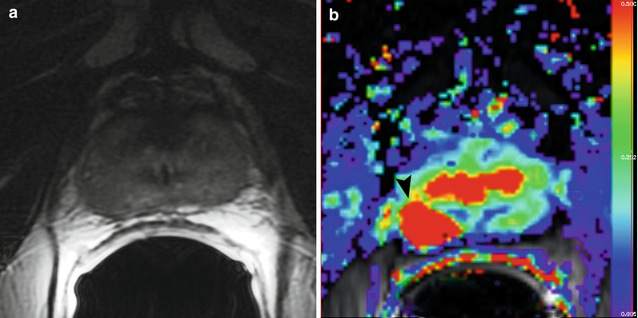
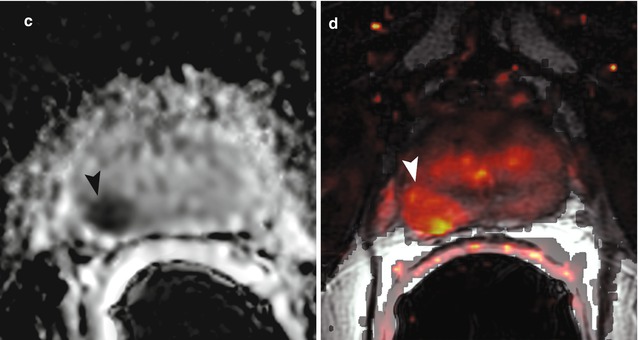
Fig. 3.6
Sixty-nine-year-old patient with biopsy-proven prostate cancer (Gleason score 3 + 4) in the right mid-gland. The cancer is not clearly visible on the T2-weighted image (a). On the parametric map of Ktrans (b), the ADC map (c, b = 0, 1,000 mm2/s), and on the fused presentation of the T2-weighted image and the Ktrans parametric map (d), the tumor (arrowheads) is clearly identifiable. Note the hypointense peripheral zone on the T2-weighted image (a) caused by post-radiation changes (Reprinted from Donati et al. [68])
For detection of lymph node and bone metastases, PET/CT with radiolabeled choline ([11C]choline) or choline analogs ([18F]choline or [18F]fluoroethylcholine) (Fig. 3.7) has been found to yield promising results in patients with recurrent prostate cancer [70–72]. Choline PET/CT can detect metastatic prostate cancer at low PSA levels and may guide local therapies such as secondary lymph node dissection [70, 71].
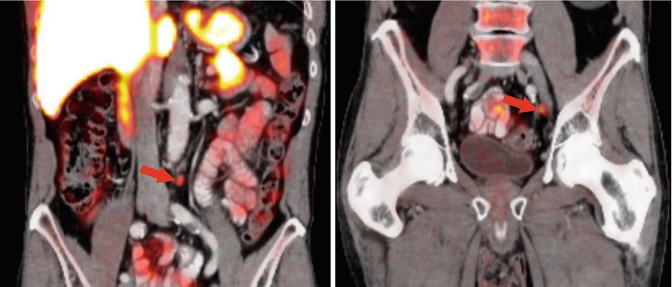
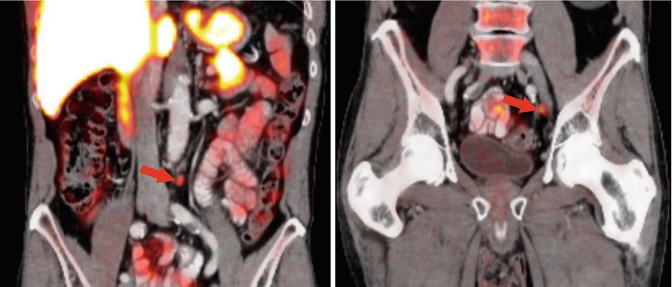
Fig. 3.7
Detection of lymph node metastases of prostate cancer (Gleason score 8) by 18F-fluoroethylcholine PET/CT. The PET/CT images show focal uptake in normal-sized lymph nodes in the left common iliac and the left paraaortic areas (red arrows)
The use of FDG-PET to monitor tumor response to therapy is well established in Hodgkin’s lymphoma (Fig. 3.8) and aggressive non-Hodgkin’s lymphomas. In these diseases, response status is now predominantly dependent on the FDG-PET findings. PET is also increasingly used in lymphomas and many solid tumors to determine tumor response early in the course of therapy.
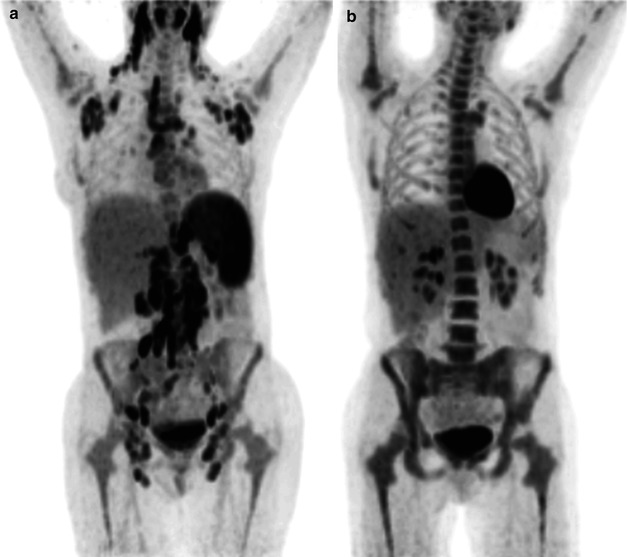
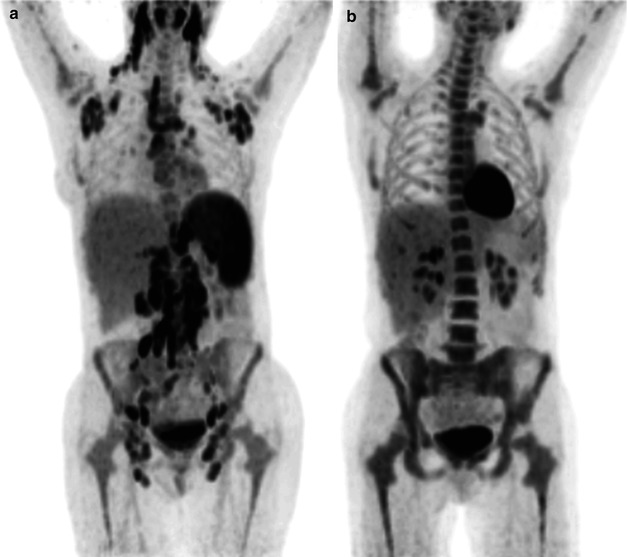
Fig. 3.8
18F-FDG-PET in Hodgkin’s lymphoma before (a) and after (b) two cycles of chemotherapy. The maximum intensity projection images demonstrate a complete metabolic response indicating a favorable response to chemotherapy
PET-guided response-adapted therapy has been most extensively studied in Hodgkin’s lymphoma and diffuse large B-cell lymphoma. Several studies have shown that tumor response after two to three cycles of chemotherapy is strongly predictive of progression-free survival [73]. Thus, treatment may be intensified in patients without a metabolic response on PET or de-escalated in patients with a favorable response on PET.
Response-adapted therapies are also being explored in solid tumors. In esophageal cancer quantitative changes in tumor FDG uptake after 2 weeks of preoperative chemotherapy were shown to be predictive of histopathologic response and progression-free and overall survival. Based on these data, a phase II study evaluated response-adapted therapy in patients with locally advanced distal esophageal cancer [74]. In patients for whom PET failed to show a metabolic response, neoadjuvant chemotherapy was stopped after 2 weeks of therapy and immediate surgical resection was performed. In contrast, patients with a metabolic response on PET received the full 3 months of preoperative chemotherapy. The results of the study indicated that response-adapted therapy is feasible and enriches the histopathologic response rate in the group of patients classified as metabolic responders. These patients demonstrated excellent progression-free and overall survival. In the group of metabolic nonresponders, progression-free survival and overall survival were not inferior to historic controls who were treated with 3 months of chemotherapy irrespective of their response on PET. Ongoing studies are evaluating whether outcome of metabolic nonresponders can be improved by changing chemotherapy early in the course of treatment (CALGB-80803, NCT01333033).
A novel approach to study tumor metabolism during therapy is MRI with hyperpolarized, 13C-labeled, metabolic substrates. For example, animal studies have suggested that by detecting changes in lactate levels, such metabolic imaging may be useful for monitoring tumor response to therapy for lymphoma and brain tumors [22, 75]. Hyperpolarized [1-13C]pyruvate has also been found to distinguish early-stage prostate cancer from late-stage prostate cancer based on lactate levels [22]. The first clinical trial of MRI with hyperpolarized [1-13C]pyruvate was recently conducted in patients with prostate cancer.
3.3 Molecular Imaging in Drug Development
Many novel cancer drugs bind to specific targets, such as protein kinase domains or cell surface antigens. It is possible to convert these therapeutics into molecular imaging probes by radiolabeling. Examples include erlotinib, a drug which inhibits EGFR and has a remarkable inhibitory effect on lung cancers with a mutated form of the EGFR kinase domain, and trastuzumab, an anti-Her-2 antibody [76, 77]. However, it can be challenging to develop imaging based on this approach because the unspecific binding of the drug may be greater than the specific binding, especially for small molecules targeting intracellular targets. For androgen and estrogen receptors, specific ligands are available that allow noninvasive imaging of receptor expression as well as monitoring of therapies that target these two classes of receptors [78–80].
3.4 Theranostics
Theranostics describes the use of a diagnostic test to assess the presence of a molecular target in order to determine the suitability–and in some cases guide the application–of a particular therapy. While the term “theranostics” is new, the concept has been used for many years in nuclear medicine for diagnosis and therapy of thyroid disorders. In fact, the first report of radioiodine treatment of metastatic thyroid cancer from 1946 used measurement of radioiodine uptake by the metastasis to guide therapy with radioiodine.
Theranostics can be practiced by performing in vitro testing and selecting in vivo therapy based on the test results [81]. The most established examples of this approach are probably HER-2 testing of breast cancer tissue before the administration of trastuzumab and determination of the presence of EGFR kinase mutations before therapy of non-small cell lung cancer with erlotinib or gefitinib [82, 83
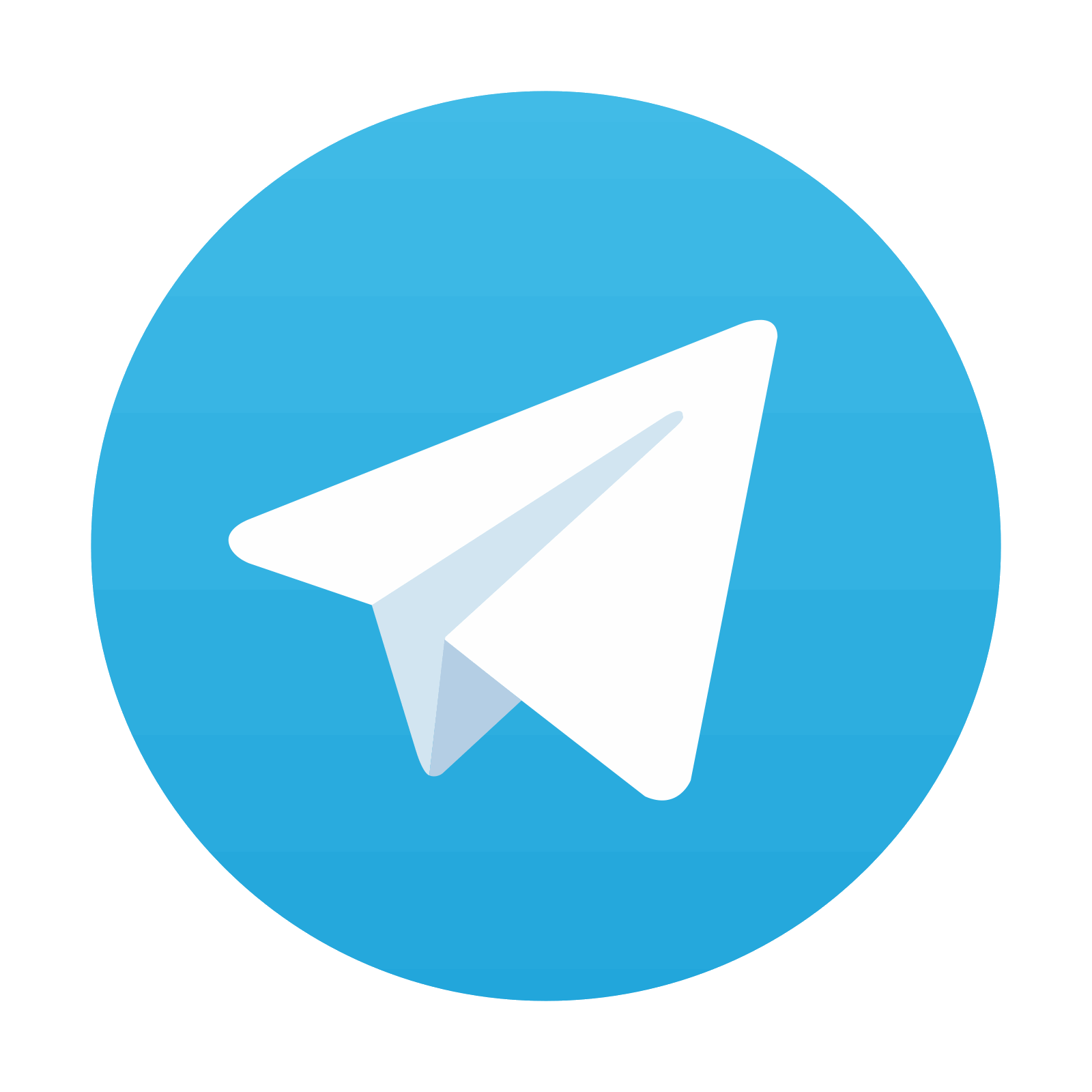
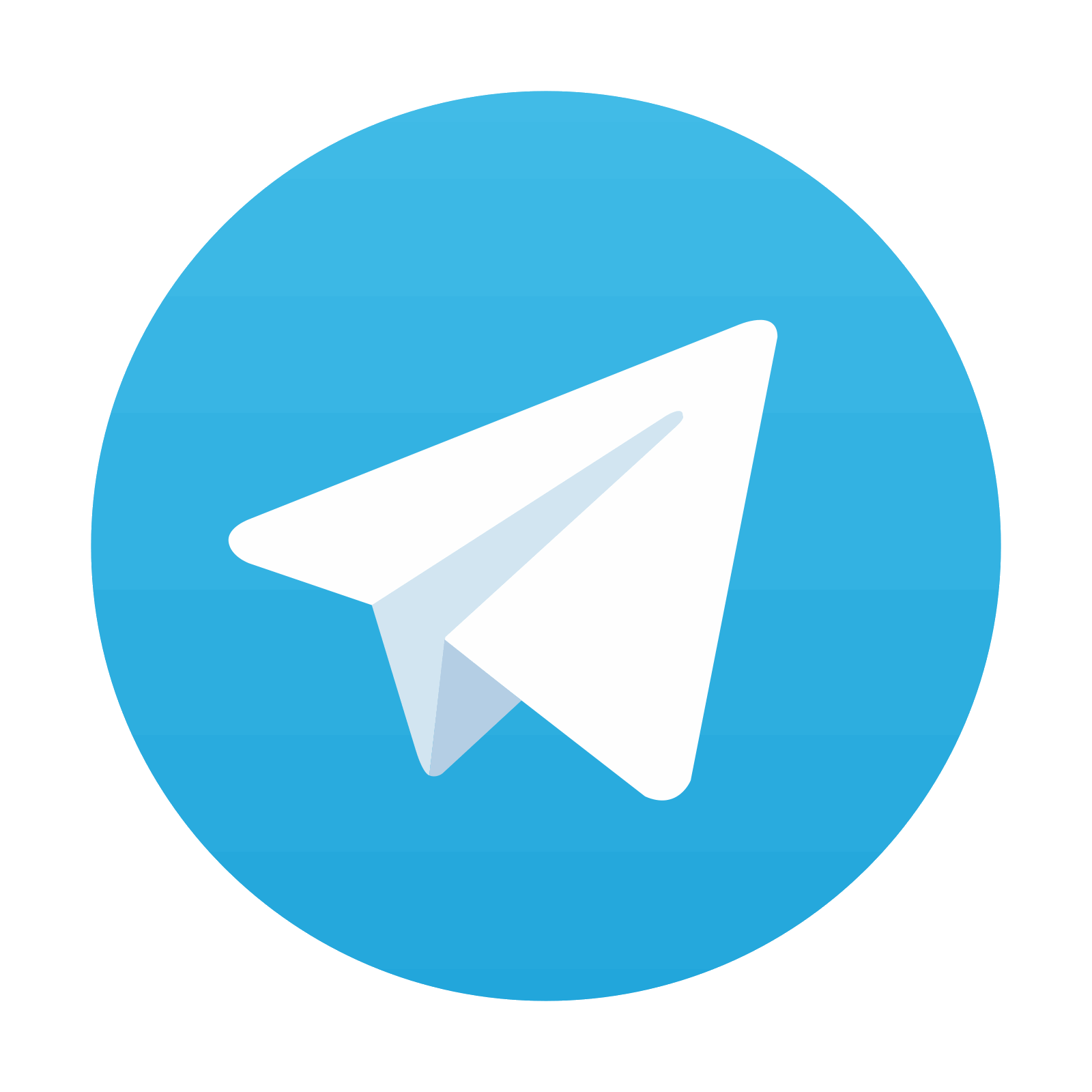
Stay updated, free articles. Join our Telegram channel
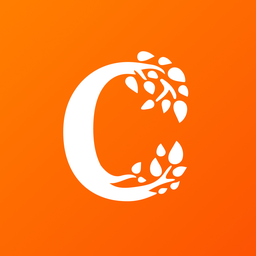
Full access? Get Clinical Tree
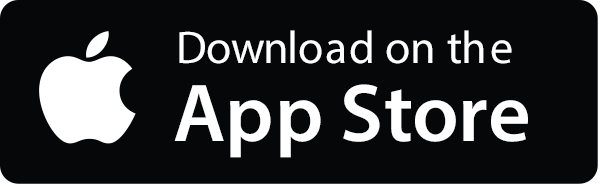
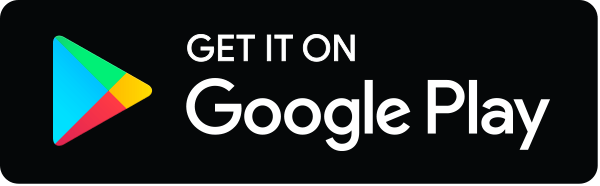