Fig. 1.
Cartoon depicting the mechanism of CEST contrast for lL-arginine dissolved in water. (a) Saturation pulses are placed either on resonance with the exchangeable guanidyl protons or off resonance and on the opposite side of the water resonance. The CEST contrast (MTR asym) is the difference between the two experiments. (b) lL-arginine dissolved in water. The guanidyl protons exchange rapidly such that the guanidyl proton saturation spreads through the network of solvent water protons.
There are two different categories of chemical exchange which can influence MR spectra: intramolecular exchange (for example helix-coil transitions of nucleic acids or the folding/unfolding processes for proteins) and intermolecular exchange (for example protonation/deprotonation processes or binding of small molecules to macromolecules). Intermolecular exchange is most relevant for MR contrast agents, as this includes the interactions of a solute molecule (or well-dispersed particle) with the bulk solvent or the source of image signal. In fact, for CEST, we are particularly interested in describing proton exchange with water because MRI is primarily a water imaging technique in the clinic. The reason that CEST is a viable mechanism for contrast in MRI is that the chemical exchange of protons acts as a “saturation amplifier.” Low concentrations of CEST agents can be detected due to the many exchanges between their protons and water protons. These exchanges magnify this signal loss as compared to the relative concentration between agent exchangeable protons and water. Robert Balaban and co-workers were the first to demonstrate this amplification and they coined the term CEST to describe this mechanism (24, 25, 27) when they were studying metabolites such as urea, ammonia, and many others. For a more detailed discussion of the mechanism of CEST, we refer the reader to a recent review of this topic (44).
There are a number of MR pulse sequences developed to acquire data on a system in chemical exchange with the resulting NMR spectra used to quantify the exchange time constants. For CEST imaging, however, the main method used is the basic saturation transfer scheme with a saturation pulse incremented across the NMR spectrum and the heights of the peaks observed to determine which spins are exchanging with the others. How does one describe the contrast mechanism for this sequence? The Bloch equations have long been used to describe the trajectory of the magnetization and can be adapted to include terms describing the physical exchange of spins. Assuming a small pool of solute protons (s) and a large pool of water protons (w) while applying B 1 along the x-axis, the Bloch equations for a two-pool proton exchange model are as follows (45):






in which
(Larmor frequency of the static magnetic field) and
(precession frequency to flip the magnetization during an rf pulse);
and
are the chemical shift differences between the saturation pulse and the solute and water resonance frequencies, respectively; M 0 is the equilibrium magnetization. Proton exchange between the two pools occurs with rates k sw (solute → water), and k ws (water → solute), and
at equilibrium.

([1])

([2])

([3])

([4])

([5])

([6])




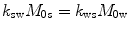
1.3 Types of CEST Agents
For MR imaging using CEST, Balaban and co-workers were the first to come up with the idea of using the saturation transfer experiment for imaging molecules with chemically exchangeable groups (24) and they demonstrated that chemical exchange between protons on metabolites and water could be detected sensitively with MR imaging both ex vivo (25) and in vivo (26) on endogenous metabolites such as urea or ammonia. A cartoon depicting the mechanism for these agents is shown in Fig. 2a. In addition van Zijl and colleagues showed that contrast agents could be constructed based on polymers containing many exchangeable protons such as polypeptides (46) or poly-nucleic acids (47) with the molar sensitivity of these agents greatly enhanced as compared to the small metabolites. In the so-called amide proton transfer (APT) imaging, Zhou et al. (48, 49) then demonstrated that this CEST effect could in fact be used to image the pH effect in the rat brain during ischemia and also highlight regions with large endogenous protein content, such as is the case in tumors in vivo. In addition and concurrently, Sherry and co-workers as also Aime and co-workers showed that exogenous agents can also be made up of complexes containing paramagnetic lanthanides (50–52) and termed PARACEST agents. These agents have ligated water or other paramagnetically shifted protons that exchange with bulk water. Two cartoons depicting two types of PARACEST agents are shown in Fig. 2b and c. More recently, Terreno and co-workers have shown that liposomes which contain paramagnetic shift agents can be used as CEST agents due to the shifting of the water in the lumen of the liposome compared to the bulk, which they have termed LIPOCEST agents (53, 54). A cartoon depicting this type of agent is shown in Fig. 3a. Other types of CEST-generating particles have been developed by Winter and co-workers (55), and more recently presented by Liu and co-workers (56), with cartoons depicting the two-exchange behavior of these particles in Fig. 3b and c.
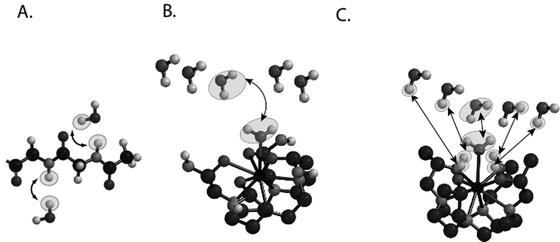
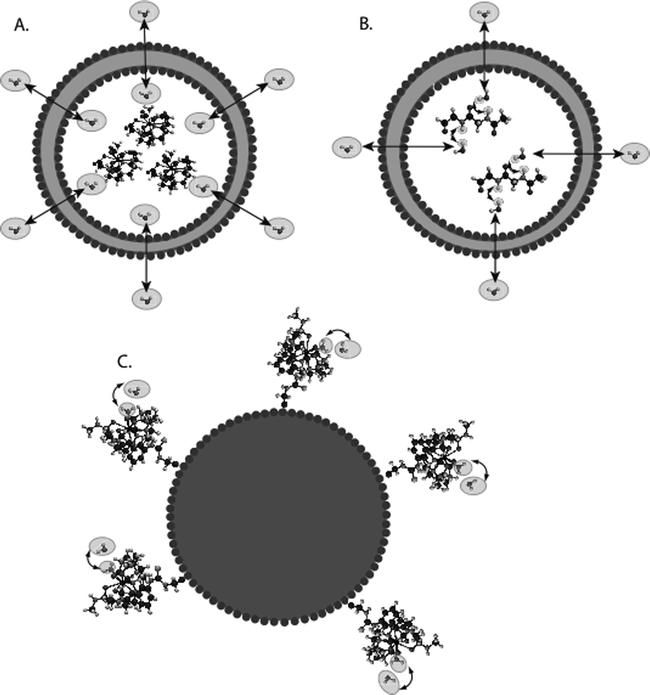
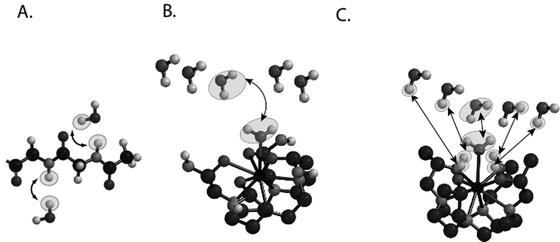
Fig. 2.
Cartoon demonstrating the three different types of CEST contrast agents. (a) DIACEST N-acetyl-Gly-Gly fragment with chemical exchange occurring between the backbone NH protons and water. (b) PARACEST Ln(DOTAm) fragment with chemical exchange occurring between ligated and free water. (c) PARACEST Ln (with chemical exchange occurring between ligated and free water and also between ligated alcohol protons and water.
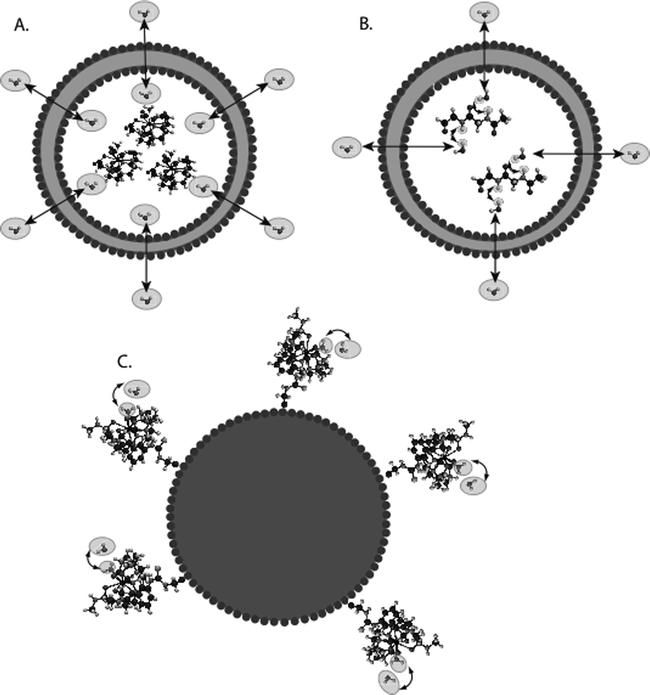
Fig. 3.
Diagram of the contrast mechanism for three different types of CEST contrast particles. (a) Traditional LIPOCEST agents, with shift agent enclosed in the liposomal lumen, and chemical exchange occurring between the interior and exterior water. (b) Two-hop LIPOCEST agents, with CEST agent (not shift agent) enclosed in the liposomal lumen, and proton exchange occurring between agent and interior water, then interior water and exterior water. (c) CEST coated particles, where the exchange occurs entirely on the periphery of the particle.
One exception to the case of using protons as CEST agents has been developed by Pines and colleagues using hyperpolarized xenon gas and molecular cages instead of water and CEST agents which are soluble. They have shown that cryptophane cages can be used to create chemical shift differences between free and encapsulated hyperpolarized xenon, with rapid exchange between the two on the timescale of the T 1 (57, 58). This exchange allows saturation transfer imaging of these cages, which they have termed HYPER-CEST. Since hyperpolarization amplifies the available magnetization by >104, the limitation of imaging xenon as opposed to water protons (used for the other agents and abundant in biological tissues) might be overcome. The set of hardware and methods we discuss in this chapter are not suitable for HYPER-CEST experiments/agents.
2 Materials
2.1 General Requirements
1.
A high-field (3 T or above) MR scanner, which can have a medium bore (89 mm) or higher with a relatively homogenous main magnetic field, fast and reliable gradient coils, and a high signal-to-noise radiofrequency (RF) coil.
2.
A set of sample containers which fit within the RF coil, typically 1 mm capillary tubes with a holder (typically plastic) to organize the containers within the coil. We have fashioned the holder from 384-well cell culture plates.
3.
For our method the most important component is the post-processing to correct the B 0 inhomogeneities present in the CEST images; as such some sort of image processing software is required and can be one of several commercial software packages, including MATLAB (Mathworks, Natick, MA), IDL(ITT visual information solutions, Boulder,CO), or others.
4.
The general lab equipment should include a water bath, pH meter, rotary evaporator (rotovap), fluorescence reader (such as the Victor V, PerkinElmer).
5.
DIACEST (such as L-arginine, Poly-lL-Lysine or others), PARACEST (such as Eu-DOTA-4AmCE or Tm-DOTA-4AmCE) or shift agent (such as Tm-DOTMA) compounds are needed to insert into the liposomes.
6.
The components of the liposomes will be required, which includes egg Phosphatidyl Choline (EggPC), cholesterol, 1,2-distearoyl-sn-glycero-3-phosphoethanolamine-N-[maleimide(polyethylene glycol)-2000](DSPE-PEG2000) and a fluorescently labeled lipid such as 1,2-dipalmitoyl-sn-glycero-3-phosphoethanolamine-N-(lissamine rhodamine B sulfonyl) (RhodPE). These can be purchased from Avanti Polar Lipids.
7.
Saline-based buffers are required for dissolving the CEST agents such as 10 mM phosphate-buffered saline (PBS) or 10 mM tris-buffered saline (TBS) or others.
8.
Concentrated solutions of NaOH and HCl will be required for titrating the solutions and chloroform will be required for dissolving the liposome components.
9.
A 50 mL round bottom flask with a few hollow glass beads are needed to perform the extended hydration of the liposomes.
10.
A liposome extruder, (such as a Liposofast-Basic extruder from Avestin) is needed to adjust the size of the liposomes. Also needed are polycarbonate filters (50–400 nm cutoff) which can be purchased from Northern Lipids Inc. Also a dynamic light scattering instrument such as the ZetaSizer from Malvern Instruments is needed to measure the hydrodynamic size of these nanoparticles.
11.
250 kDa cutoff PVDF dialysis tubing (Spectrum Laboratories, Inc.) and a 2 L beaker are required for separating the liposomes from unencapsulated CEST agent.
2.2 Liposome Sample Influences on CEST Contrast
There are many different ways to prepare DIACEST, PARACEST or LIPOCEST agents. In this chapter, we will describe how to prepare DIACEST liposomes, with the advantage of these being that they can be readily prepared using simple lab equipment, extruded to a variety of sizes (from ∼100 nm to ∼800 nm) and produce sufficient CEST contrast for detecting these particles in vivo. These particles are depicted in Fig. 3b.
1.
CEST contrast for these liposomes will vary in part as a function of the permeability of the phospholipid bilayer. This permeability is affected by the lipid/sterol content and also, if cholesterol is added, the relative percentage of cholesterol (mole percentage) to the other components.
2.
The surface area–volume (SA–V) ratio of these liposomes also influences the CEST contrast. However the size of the liposome (and resulting SA–V ratio) also affects the biodistribution of the particles so there might not be a choice as to what size liposome should be prepared, even if the maximum contrast is not achieved by this size.
2.3 MRI Pulse Sequences
The following pulse sequences should be available.
1.
A multi-slice imaging sequence such as fast spin echo (FSE), or rapid acquisition with relaxation enhancement (RARE) with the ability to place a saturation pulse or pulses in front of the imaging sequence and also with the ability to change the saturation pulse time, field strength, and frequency offset such as that found in the magnetization transfer (MT) module on Bruker scanners.
2.
A localized spectroscopy sequence, such as point-resolved Spectroscopy (PRESS).
3.
A fast 3D scout image sequence, such as fast low angle shot (FLASH).
2.4 In Vitro B0 Correction Algorithm
B 0 inhomogeneities, if left uncorrected, can erroneously increase or reduce CEST contrast (59, 60) which presents complications for the practical imaging of these agents. Recently, it was shown that the z-spectra produced by incrementing a low-power, short saturation pulse can be used to map the absolute water frequencies of each voxel in an image, an approach termed water saturation shift reference (WASSR) mapping (61). Since the saturation pulse is short and weak, both CEST and conventional magnetization transfer (MT) contributions to the z-spectra are minimized and only direct water saturation spectra are obtained. The CEST-weighted images can then be corrected in a pixel-by-pixel manner using the WASSR absolute frequency map and the interpolation. These frequency-weighted images can be used to determine the type of CEST agent contained in each voxel in the image, as shown previously (62, 63).
3 Methods
3.1 Liposome Preparation (see Note 2)
1.
Dissolve CEST agent (such as L-arginine (Larg)) in 10 mM phosphate-buffered saline (PBS) (or other buffer such as tris-buffered saline (TBS)) at a concentration of 14 mM and titrate to pH 7.4 using HCl or NaOH.
2.
The liposome components will have a molar ratio of eggPC:Chol:DSPE-PEG2000:RhodPE of (46.6:46.6:5:1.8). These are all dissolved in chloroform individually, then transferred to a 50 mL round bottom flask using volumes of 0.47 /0.140 /0.27 /1.0 mL respectively, and then stirred manually in a chemical fume hood.
3.
The chloroform is evaporated in a rotovap with the water bath set to 50°C. As a result, the lipids form a thin film that coats the side of the flask.
4.
Add a few hollow glass beads and hydrate the lipid thin film with 1 mL of the solution containing dissolved CEST agent. Stir the mixture manually until lipids are no longer visible.
5.
Anneal for 2 h in a 55°C water bath and stir occasionally.
6.
20 μL of the solution is put aside for fluorescence measurement of the concentration of the liposomes. The remaining solution that contains liposomes is then extruded to reduce the liposome size using 400, 200, 100, and 50 nm cutoff membranes, using two filters. The liposomes are passed through the 400 nm polycarbonate membranes 21 times first, then depending on the desired liposome size, are passed through the 200 nm and 100 nm membranes also using 21 passes through the filters.
7.
After the liposomes are extruded, the unencapsulated agent is removed by dialysis overnight in 1× PBS buffer using 250 kDa cutoff PVDF dialysis tubing (Spectrum Laboratories, Inc.).
8.
The size and concentration of the liposomes are measured using dynamic light scattering and fluorescence, respectively.
3.2 General MRI Protocol (see Note 3)
1.
The phantom should be centered in both the RF coil of the imaging probe and the magnet bore through adjustment of the position of the imaging probe.
2.
The RF coil of the imaging probe is then tuned and matched according to the scanner’s 1H Larmor frequency (see your scanner manual for details of this procedure).
3.
Next, a scout image is acquired (using for example tripilot RARE, MSME (multi-slice multi-echo), or FLASH on Bruker small animal scanners), with a sufficient field of view (FOV) so that three views from the scout (XZ, YZ, XY) capture the whole phantom.
4.
The pulse sequence parameters should be calibrated including resonance frequency offset, pulse power for 90° and 180° flip angles, and receiver gain; and the magnet can be shimmed (although not entirely necessary with the B 0 correction routine we will employ in Section 3.3).
5.
The desired slice for the CEST experiment should be chosen, based on considerations from the scout image such as the distance to the center of the coil or position of air bubbles in the sample tubes. Due to the fact that CEST imaging employs a long saturation pulse, a single-slice approach is typically preferred in a CEST acquisition to save time. A single-slice RARE sequence without turning on the saturation pulse is then tested to ensure that the defined single-slice geometry sufficiently covers the region of interest.
3.3 Determining B0 Inhomogeneity
3.3.1 Shimming
CEST imaging is often (though not always, as demonstrated by Vinogradov and co-workers (64)) a difference imaging technique dependent on the frequency of the solvent and, as such, either good shimming or accurate B 0 maps are crucial to measure CEST contrast. Automatic shimming procedures are available on most scanners (generally restricted to first-order shims), or the shimming can be improved further through manual shimming. The shimming should be performed on a slice with the geometry similar to or equivalent to that used for the CEST imaging and the magnetic field should be shimmed to maximize the signal (see Note 4).
3.3.2 Estimating B0 Inhomogeneity Over the CEST Slice
The range of frequencies encompassing the water signal can be determined using the point-resolved spectroscopy (PRESS) sequence without water signal suppression with the voxel geometry approximating the CEST imaging (or up to 50% larger in slice thickness). The typical acquisition parameters used are TR/TE = 2000/20 ms; spectrum acquisition size = 8192; sweep width = 10,080 Hz (25 ppm at 9.4 T); and NA = 1. The full spectral width of the water peak is then used to determine the range of B 0 inhomogeneity, after processing the spectrum (including FFT, baseline correction, apodization, etc.).
3.3.3 WASSR Acquisition
The WASSR images are acquired by adding a saturation pulse (called the magnetization transfer module on Bruker scanners) in front of an FSE or RARE sequence. The saturation pulse we typically use is a 200–500 ms continuous wave (CW) pulse with B 1 = 0.5 μT (21.3 Hz, see Note 5). The saturation pulse is typically scanned across the entire B 0 inhomogeneity range we determine using PRESS (typically from −1 ppm to +1 ppm with respect to water for twenty 1 mm capillaries in a 20 mm RF coil) using a 0.1 ppm increment between each acquisition. The typical imaging parameters used are acquisition bandwidth = 50 kHz; single slice; 1 mm slice thickness; TE = 6 ms; TR = 1500 ms; RARE factor = 16; FOV = 20 × 20 mm (see Note 6); and the matrix size is set to 128 frequency-encoding steps and 64 phase-encoding steps. The total acquisition time for generating an absolute B 0 map varies from 3 to 10 min. The error of B 0 estimation is under the hertz level for each pixel at a reasonable signal-to-noise ratio (SNR) (i.e., SNR/pixel > 15), using the parameters listed above.
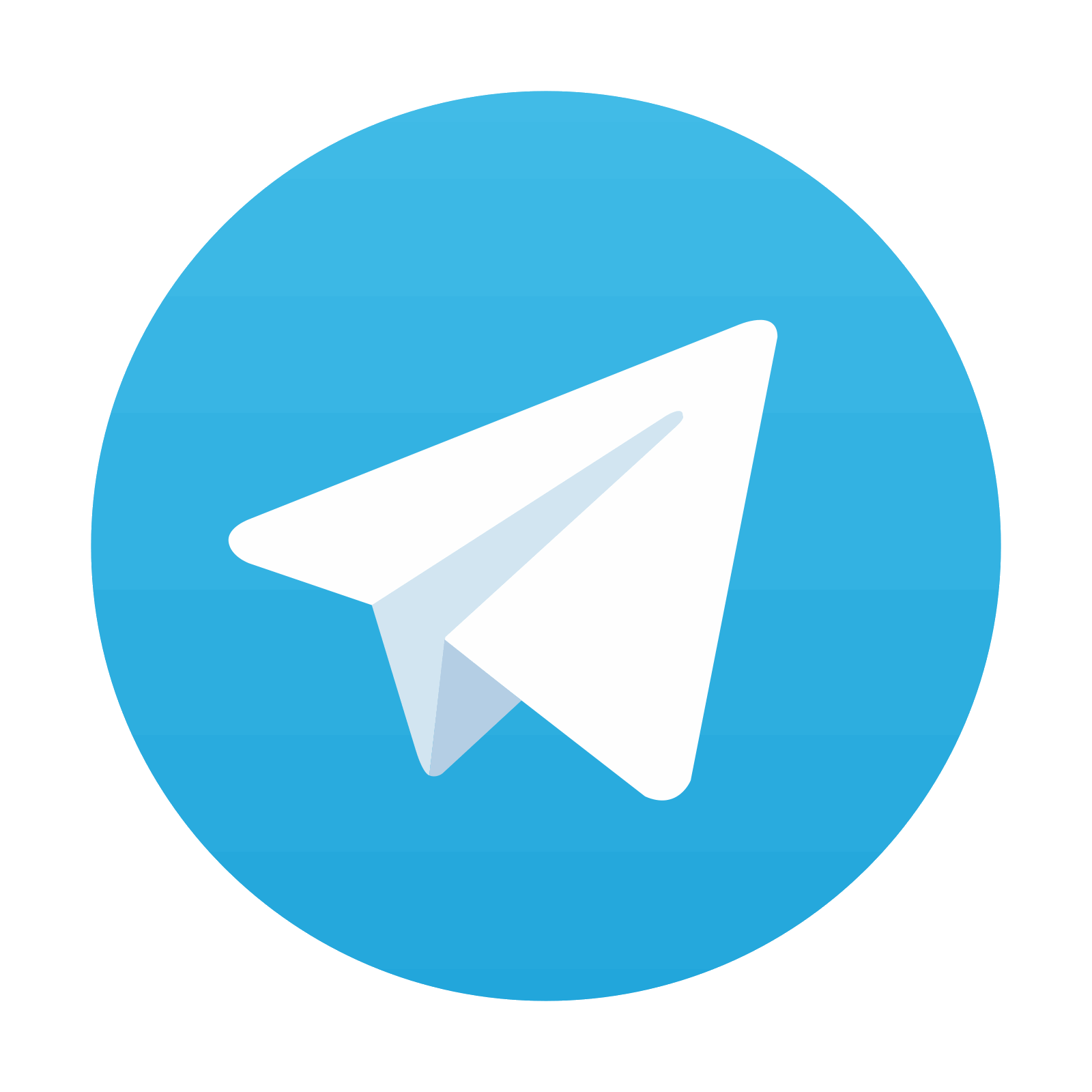
Stay updated, free articles. Join our Telegram channel
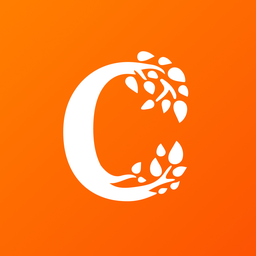
Full access? Get Clinical Tree
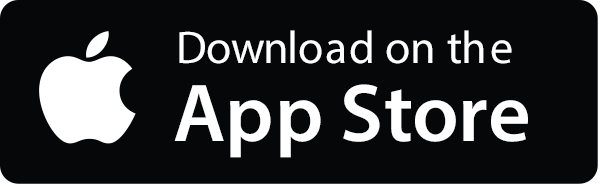
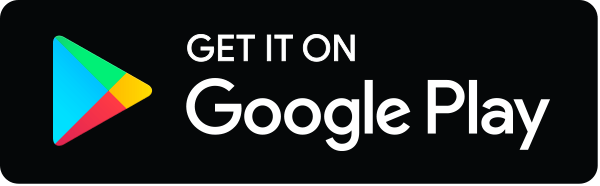