© Springer International Publishing Switzerland 2017
Tommaso Scarabino, Saverio Pollice and Teresa Popolizio (eds.)High Field Brain MRI10.1007/978-3-319-44174-0_1313. Setting the Report and Support of the Functional Findings
(1)
Department of Neurosciences, Imaging and Clinical Sciences, Institute of Advanced Biomedical Technologies, University “G. d’Annunzio”, Chieti, Pescara, Italy
13.1 Functional Data in Clinical Practice and Research
Advanced MRI, including functional MRI (fMRI), has become an increasingly useful tool and ever more used, not only in research field but also in clinical practice. Nowadays, MRI is no longer considered just a qualitative diagnostic imaging but also a quantitative tool.
Advanced MRI techniques allow a noninvasive method for investigating normal and pathological tissues. Quantification of metabolite rates, perfusion parameters, and water diffusivity indices opened new scientific applications with a shift from qualitative to quantitative MRI [1, 2].
In the fMRI experiment, data are used to detect, in an indirect way, the changes in specific brain areas of neuronal stimulation-related activity. The fMRI signal is effect related to the local variations in blood flow that follow the changes in neural activity. Although signal intensity varies of only about 1 percent, fMRI allows to recognize brain areas involved in motor, sensorial, and cognitive functions.
Thanks to the greater signal-to-noise ratio, 3 T MRI provides more reliable and more reproducible data than 1.5 T MRI [3]. In clinical practice, combined data, obtained by means of advanced MRI techniques and fMRI, are considered as “potential” biomarkers for the assessment of both microstructural (and/or microvascular) changes and anatomo-functional reorganization of brain connectivity, induced by focal or degenerative brain diseases.
Diffusion-weighted imaging (DWI), diffusion tensor imaging (DTI), magnetic resonance spectroscopy (MRS), and perfusion-weighted imaging (PWI) arterial spin labeling (ASL) provide quantitative, reliable, and reproducible information about microvascularity, neoangiogenesis, metabolism, necrosis, and cellularity of brain masses [4].
Since fMRI began in 1991, the number of people, papers, and abstracts related to fMRI has been increasing. Because fMRI is based on the blood oxygenation level-dependent (BOLD) effect, it does not directly record neural activity. Nevertheless, due to the excellent spatial and time resolution, fMRI is progressing in technology and methodology and increasingly used to investigate human brain function.
Functional connectivity (FC), defined as “temporal correlations between spatially remote neurophysiological events,” is the most recent challenge of the fMRI technique. Resting-state fMRI (rs-fMRI), seen as a constant condition without sensorial stimuli or significant behaviorally salient events during fMRI recording, may be altered in dementia as well as in aging-related cognitive disorders.
Changes in the brain structure and function are well recognized in Alzheimer’s disease (AD). Alterations of FC have been observed in patients affected by AD and mild cognitive impairment (MCI) and in subjects who had subjective cognitive decline (SCD). Among the rs-fMRI observations, the so-called default mode network (DMN), the most active neuronal network at rest and in the absence of a stimuli during fMRI experiment has been particularly studied because alterations to its FC were found in AD and MCI patients [5, 6]. These studies helped to understand the neural substrates in normal and pathological aging brain.
A challenge for clinical application of the advanced and fMRI techniques is closely related to the reliability and reproducibility of the results, which follows a standardization of acquisition techniques and data analysis.
13.2 Standardization of Data Handling
13.2.1 Image Acquisition
In advanced MRI protocols, brain images are acquired by using different sequences, some of which are critical for structural high-resolution and functional studies.
In general, advanced imaging protocols must include morphological T1-weighted sequences with high spatial resolution and isotropic voxel; currently, for this purpose whole-head 3D gradient-echo (GE) sequences, which though have different owner acronyms, are all of the type GE spoiled (SPGR, mPRAGE, 3D GE, etc.) and can be obtained in 5–6 min. Isotropic high-resolution structural images are used for voxel-based morphometry (VBM) and cortical thickness quantification of the whole brain or specific brain areas in neurodegenerative diseases, such as dementia [7]. These images are also frequently used as anatomical substrate for co-registration of BOLD-fMRI signals and spatially localization of eloquent areas in the presurgical brain mapping.
An ideal and desirable voxel spatial resolution for morphological T1-weighted sequences, obtained by using a 3-Tesla MRI system, is 1×1×1 mm or less (e.g. 0.8 × 0.8 × 0.8–1 mm).
T2*-weighted sequences, acquired with echo planar imaging (EPI) technique, are basically used in all BOLD-fMRI, PWI, DWI, and DTI, respectively; functional and microstructural studies, as well as morphological T1-weighted sequences, are used for high-resolution structural quantitative studies (VBM, cortical thickness). When using EPI sequences with a 3-Tesla MR system, in the scan time relative to each TR value (e.g., 1 s), up to 30 gapless, whole-head, 3 mm thickness or less, T2*-weighted images can be acquired.
MRS studies are basically obtained using specific sequences for the acquisition of the spectroscopic signals. Single voxel spectroscopy (SVS) receives spectra from a single voxel only; the signal-to-noise ratio (S/N) is generally high but limited to a circumscribed area. Multivoxel spectroscopy (MVS) explores larger brain regions, but S/N is lower than the SVS for the smaller voxel size. In SVS signal acquisition can be acquired with two different sequences. Basically, in PRESS (Point RESolved Spectroscopy) technique, the signal from the voxel of interest is a spin echo; in STEAM (Stimulated Echo Acquisition Mode) technique, the stimulated echo, corresponding to the S/N from the voxel of interest, is obtained from the cumulated effect of three pulses. The value of time echo (TE) used for PRESS and STEAM sequences determines the number of detectable metabolites on spectroscopic spectrum. When a long TE is selected (e.g., 144 or 288 ms), a more number of metabolites are visible, but S/N is lower than short TE (e.g., 30 ms or less), where a greater number of peaks are visible in the spectrum [8].
Even today, the major limitation is the lack of standardized imaging protocols and sequence parameters (e.g., TR, TE, FA, TI, slice thickness), both in clinical and research studies. Lack of standardization not only affects the reproducibility of the results, but mainly the routinely use of advanced MRI outcomes.
13.2.2 Processing of the Stimulus
In fMRI experiments, standardization of the procedures includes also the modalities of stimuli administration, scan paradigms, and data recording. Using standardized fMRI protocols, the starting point is to acquire “some” good advanced MRI data, in both high-resolution structural MRI and fMRI, task or resting state what they are. Before statistical analysis and signal detection is performed, it is necessary to convert and to improve the signal quality by preprocessing the raw data. In the preprocessing, different function must be implemented to obtain reliable data. Slice scan time correction, motion correction, temporal filtering, and spatial smoothing functions must be defined and applied preliminarily when a group analysis is required. Additional preprocessing (e.g., physiological noise correction, storm filtering, band pass) is also recommended before executing group data analysis.
In functional and effective connectivity measures, processing of brain activations is generally based on independent component analysis (ICA) and/or seed-based methods, in order to identify and quantify brain interregional relationships. Both ICA-derived and seed-based connectivity measures are characterized by some advantages and disadvantages, but a direct comparison of the results obtained from the two methods is not trivial. Also different paradigms, designed for brain stimulation, can influence qualitatively brain responses and qualitatively data recording. The major paradigms for fMRI analysis are univariate (hypothesis-driven), multivariate (data-driven), and voxel-based approach. FC networks can be detected from event-related designed paradigm, most often based on trials under stationary condition, or from block design paradigm, considered locally stationary and based on block duration [9].
13.2.3 Data Analysis
There are several techniques for interrogation of brain functions and as many available programs for analyzing advanced MRI data, each of which can have a large influence in determining the sensitivity, specificity, reliability, and flexibility of the experimental protocols. There are a large variety of software packages for data analysis, although not all of these single software packages can provide all necessary requirements for both structural and functional processing. fMRI data can be preprocessed and analyzed by using several platform operating systems and a number of software packages, e.g., SPM (statistical parametric mapping), BrainVoyager analysis, AFNI (analyses of functional software neuroimages), FSL (FMRIB Software Library), FreeSurfer, and many others as valuable software [10]. Biomedical image analysis software is a multimodal analysis tool, developed to analyze and visualize the structural and functional brain imaging data recorded from neuroimaging techniques and technologies (fMRI, DTI, EEG, MEG, TMS, PET, etc.). Nowadays, this software allows statistical data analysis based on general linear model (GLM), transformation of anatomical and functional scans into stereotaxic (Talairach-Tournoux) coordinate, automatic co-registration of functional data with high-resolution 3D anatomical data sets, and advanced methods for automatic brain segmentation, surface reconstruction, cortex inflation, and flattening. [11].
After being considered for years, the “future technique,” MRS, finally, in the last decade, has become a valuable tool in clinical practice and research, for in vivo analysis of the main brain metabolites. Certainly, it has had great influence on the success of MRS techniques in the progress in signal processing. Currently, different valuable software packages are available for processing and quantitation of MRS data. Automated, proprietary software provided by the scanner manufacturers has the advantage of being fast in the analysis of MRS data, but when the S/N ratio is not high, there may be a lower resolution of the spectra for certain metabolites. A number of semiautomatic dedicated software packages are now available for MRS data generation, either in frequency (e.g., LCModel) or time domain (e.g., jMRUI). Commercially or freely available software packages take longer time for data processing, but S/N ratio is higher than automated software [8]. In general radiologists are more confident with software provided by the scanner manufacturers, because it is easy to use and less time-consuming, and the raw data should not be processed remotely.
As for MRS also PWI and DTI techniques require dedicated software packages for processing the raw data. Most of the radiologists prefer a fast processing of raw data at the end of MRI exam by means of automated software package provided by the scanner manufacturer. But many powerful software packages are freely or commercially available for a more accurate data processing (e.g., FreeSurfer platform and dedicated function included in the package like TRACULA (TRActs Constrained by UnderLying Anatomy)) and for automatic or semiautomatic reconstruction of WM tracts from diffusion-weighted images [12]. Several software packages are also available for calculation of quantitative PWI parameters (CBF, CBV, MTT), but in clinical practice radiologists feel more confident using automatic software provided by the manufacturer of the MRI system, because it is less time-consuming and easy to use even during the clinical activity. More powerful and sophisticated commercially or freely available software packages are fruitfully used for clinical research and require dedicated staff, specifically trained in order to fully exploit their potential. Given the differences of data processing and the wide range of software for data analysis, advanced MRI data, especially from fMRI, still has many limitations for clinical use.
In summary, as for the image acquisition, also for data analysis, there is a lack of standardization, which makes it difficult to compare different results; in some cases it may also notice a variability of the results within the same laboratory, for which it is always recommended the preliminary standardization of procedures and the development of its own database of reference values, both for normal and clinical categories, based on large populations of normal subjects and clinical series, as well as the comparison with scientific literature.
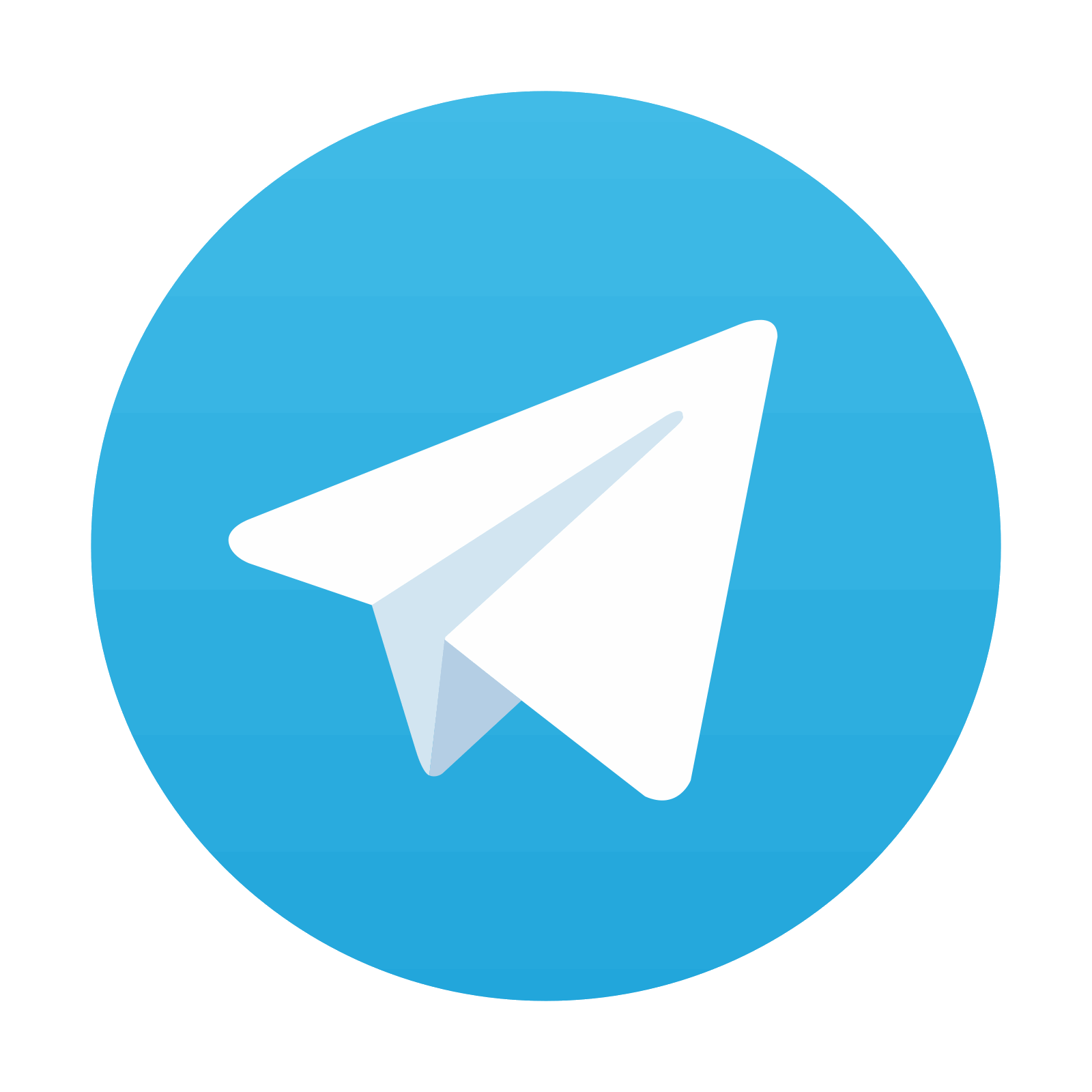
Stay updated, free articles. Join our Telegram channel
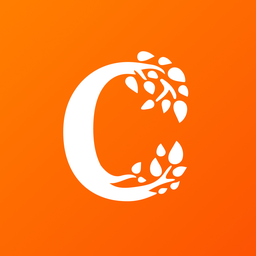
Full access? Get Clinical Tree
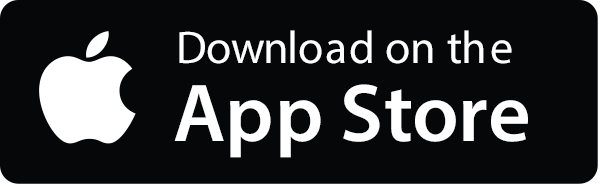
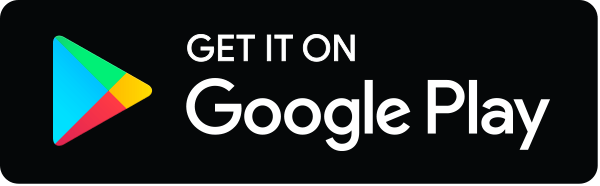