1976
1986
1992
Ophthalmic
17
17
17
Fetal imaging
46
94 (104 %)
720 (667 %)
Cardiac
430
430
720 (67 %)
Peripheral vessel
720
720
720
It is interesting to observe from Table 1.1 that, for fetal imaging, the I SPTA was allowed to increase by a factor of almost 16 from 1976 to the most recent values in 1992, yet, as will be described below, all epidemiological information available regarding fetal effects predates 1992. A further remarkable fact is that intensity for ophthalmic examination has not changed from the original 17 mW/cm2, a value approximately 42.5 times lower than the present allowed maximal value for fetal scanning. Furthermore, pelvic imaging (abdominal or transvaginal) is not specified in Table 1.1.
Tissue Characteristics
When the ultrasound wave travels through a medium, its intensity diminishes with distance [14]. Biological tissues are nonhomogeneous and weakening (attenuation) of the signal results from absorption and scattering, as well as reflection. Absorption is the sound energy being converted to other forms of energy, and scattering is the sound being reflected in directions other than its original direction of propagation. Since attenuation is proportional to the square of sound frequency, it becomes evident why higher-frequency transducers have less penetration (but better resolution). Acoustic impedance can be described as the opposition to transmission of the ultrasound wave. It is proportional to the velocity of sound in the tissue (estimated at 1,540 ms/s, see above) and to the tissue density.
Instrument Outputs
Publications of various instruments outputs are available [15]. From a clinical standpoint, there is no easy way to verify the actual output of the instrument in use. In addition, each attached transducer will generate a specific output, further complicated by which mode is being applied [16]. When comparing modes, the I SPTA increases from B-mode (34 mW/cm2, average) to M-mode to color Doppler to spectral Doppler (1,180 mW/cm2). Average values of the temporal averaged intensity are 1 W/cm2 in Doppler mode but can reach 10 W/cm2 [17]. Therefore, precaution is needed when applying this mode. Most measurements are obtained from manufacturers’ manuals, having been derived in laboratory conditions which may be different from real-life clinical conditions [18]. Furthermore, machine settings which are under the control of the clinician can alter the output. For instance, the degree of temperature elevation is proportional to the product of the amplitude of the sound wave by the pulse length and the PRF. Hence, it is evident why any change (augmentation) in these properties can add to the risk of elevating the temperature, a potential mechanism for bioeffects. The three important parameters under end-user control are the operating mode (including choice of transducer), the system setup/output control, and the dwell time.
1.
Scanning mode: as mentioned above, B-mode carries the lowest risk and spectral Doppler the highest (with M-mode and color Doppler in between). High pulse repetition frequencies are used in pulsed Doppler techniques, generating greater temporal average intensities and powers than B- or M-mode and hence greater heating potential. In spectral Doppler, the beam needs to be held in relatively constant position over the vessel of interest, which may add to the risk of a larger increase in temporal average intensity. Naturally, transducer choice is of great consequence since transducer frequency will determine penetration, resolution, and field of view.
2.
System setup: starting or default output power is very often high to allow better imaging, and end users will, generally, keep it as such, mostly out of lack of concern for bioeffects. Excellent, diagnostic images can be obtained at lower output powers (see Fig. 1.1). Recently major manufacturers have responded to requests from involved individuals and are now offering a low output power in Doppler as their default. Only if needed can the end user increase the power. Fine-tuning performed by the examiner to optimize the image influences output but with no visible effect (except if one follows TI and/or MI displays, see below). Controls that regularize output include focal depth (usually with greatest power at deeper focus but, occasionally on some machines, with highest power in the near field), increasing frame rate, and limiting the field of view (for instance, by high-resolution magnification or certain zooms). In Doppler mode, changing sample volume and/or velocity range (all done to optimize received signals) changes output. A very important control is receiver gain. It often has similar effects to the above controls on the recorded image but none on the output of the outgoing beam and is, therefore, completely safe to manipulate. In addition, over the years, as seen in Table 1.1, output of instruments has increased [17]


Fig. 1.1
Color (a) and spectral (b) Doppler of the corpus luteum. Please note the low TI (0.3 and 0.4, respectively) and MI (0.6 and 0.5, respectively) in both images
3.
Dwell time is directly under the control of the examiner. It is the time during which the ultrasound beam remains at the same point in the tissue. Interestingly, dwell time is not taken into account in the calculation of the safety indices (TI and MI) nor, in general, until now, reported in clinical or experimental studies. Directly associated with dwell time is examiner experience in terms of knowledge of anatomy, bioeffects, instrument controls, and scanning techniques, since, presumably, the more experienced the examiner, the less scanning time is needed.
Ultrasound Bioeffects
Why is this even an issue? The average ultrasound professional (and the lay people) will state that ultrasound is obviously safe and that it is not X-rays [19]. Ultrasound, similarly to any sound, is a form of energy. The waveform has positive and negative pressures (see above). When such a waveform travels through any tissue, some of this energy is transformed into heat (thermal effects), and some may cause movements of tissues or membranes as well as some more complex mechanical results (nonthermal or mechanical effects). Cavitation is one of these nonthermal effects. This refers to reaction of small gaseous bodies (bubbles) when exposed to an ultrasound field [20]. In inertial cavitation (formerly known as transient cavitation [21]), the bubble changes volume (expands under negative pressure and contracts under positive pressure) until the vibration amplitude of the bubble wall increases so much that the bubble implodes. This implosion generates highly localized shock waves and is also associated with extremely high local temperatures, up to 10,000 K [22]. This is localized on a tiny area and for a very brief moment, and no heat is actually exchanged (adiabatic reaction). However, in addition to the temperature elevation, the implosion may result in the generation of free radicals such as hydroxyl radicals and hydrogen [23]. If the bubble does not collapse during the ultrasound exposure, the condition is referred to as stable cavitation with the bubble oscillating as the waveform progresses. Absence of cavitation foci (gas bubbles), as is the case in fetal lungs and bowels and, presumably, in the vicinity of the ovary and the developing follicle, renders this phenomenon extremely improbable.
These effects occur whenever a tissue is insonated, thus the designation bioeffects [24]. Many in vitro models, such as cells or tissue cultures, have been used to investigate ultrasound bioeffects and to gain a better understanding of the possible mechanisms of interaction between ultrasound and biological tissue [25]. Studies on relatively simple nonmammalian organisms, such as insects, amphibians, and avians, are helpful in understanding the mechanisms of interaction between ultrasound and biological systems [26]. However, from a clinical standpoint, bioeffect studies of mammalian species are of more relevance. Most of these studies were performed on small rodents, such as mice or rats. The extrapolation of experimental results to humans can be difficult, at best. A very comprehensive, albeit dated, review of the effects of ultrasound on mammalian development was prepared by Sikov [27]. He evaluated bioeffects depending on gestational age and thus attempted to extract information on the relation between exposure parameters and stage of development at exposure Experimental studies indicate that intact mammalian systems (in vivo) do not show a significant rise in temperature when exposed to pulsed imaging equipment [27–29]. However, peripheral vessel pulsed and continuous-wave (CW) Doppler equipment, when used for a relatively long time (1–10 min), may be an exception [30, 31]. Therefore, Doppler should be used with care, especially during applications in which Doppler is used for the assessment of blood velocities in ovarian vessels in ART and studies of the first trimester fetus [32]. Reports on the use of new technologies, such as three- and four-dimensional (3D/4D) ultrasound, are beginning to appear in the art literature [33], but these do not appear to expose tissues to higher levels of acoustic energy [34].
The Output Indices
Because of the two main mechanisms (described above) involved in bioeffects of ultrasound, a Standard for Real-Time Display of Thermal and Mechanical Indices on Diagnostic Ultrasound Equipment, generally known as the Output Display Standard or ODS, consisting of two indices—thermal (TI) and mechanical (MI)—was implemented in the USA around 1992–1994 [11, 13, 22]. Secondary to end users’ desire for better imaging and as a result of discussions that involved the FDA, the AIUM, and the National Electrical Manufacturers Association (NEMA), in 1994 the FDA revised its guidance on diagnostic ultrasound 510(k) submissions to allow the use of the MI in place of the ISpPa in determining substantial equivalence of devices. This revision assumes that on-system displays of numerical indices, including MI and TI, will inform the user about the potential for either thermal or nonthermal bioeffects associated with the actual examination settings of the imaging system. This enables the clinician to increase acoustic power output beyond the existing FDA guidelines when clinically warranted (see Table 1.1). Before the 1994 FDA revision, such an increase was not possible. The maximum available acoustic output was limited by the manufacturer’s software, which would not allow the output to exceed FDA guidelines for maximum exposure. It must be stressed that with the implementation of the ODS, diagnostic ultrasound systems can have a higher output limit. With the higher limits comes the potential for increased risk to the patient, so the clinician must make a careful risk/benefit analysis. Therefore, the purpose of the ODS is to help the clinician implement the ALARA (as low as reasonably achievable) principle and minimize the potential for bioeffects. A very important aspect of the implementation of the ODS as explicitly emphasized in the original recommendations for adoption was education of the end users about bioeffects of ultrasound and safety-related issues. This particular goal appears to not have been very successful as indicated by the fact that 70–80 % of end users worldwide know very little about bioeffects and the safety indices [35– 38]. Furthermore, sonographers and ob/gyn residents and fellows in the USA seem to be similarly unfamiliar [39, 40].
The thermal index (TI) provides some indication of potential temperature increase, and the mechanical index (MI) provides indication of potential for nonthermal (i.e., mechanical) effects [22, 25, 41]. The TI is the ratio of instantaneous total acoustic power to the acoustic power estimated to be required to increase tissue temperature by a maximum of 1 °C. It is an estimate of the maximal temperature rise at a given exposure. It is not a measurement of the actual or assumed temperature. Some correlation exists with temperature rise in degrees Celsius, but in no way does TI allow an estimate or a guess as to what that temperature change actually is in the tissue. There are three variants: TI for soft tissue (TIS), for early pregnancy when ossification is minimal; TI for bones (TIB), to be used when the ultrasound beam impinges on bone, at or near the beam focus, such as late second and third trimesters of pregnancy; and TI for transcranial studies (TIC) when the transducer is essentially against bone, mostly for examinations in adult patients. In ART, TIS is recommended. These indices were required to be displayed if equal to or over 0.4. There are several issues with TI, in particular the fact it does not take exposure time into account. Thus, several authors have suggested modifications or frank changes in the way thermal effects can be assessed [42–44].
The MI has been developed as an on-screen indicator of the potential for nonthermal damage or cavitation-like phenomena related to B-mode operation. MI is inversely proportional to the center transducer frequency, i.e., the higher the frequency (as is used in ART), the lower the risk of mechanical effects. It is important to know that the MI is not based on actual in situ measurements. It is a theoretical formulation of the ratio of the pressure to the square root of the ultrasound frequency. Both the TI and MI can and should be followed as an indication of change in output during the clinical examination.
A complicating factor is uncertainties in TI (and MI) calculations. The error may be a factor of 2 or even 6. It is usual to consider a factor of 2 in risk evaluation. Hence, a TI of 2 may indicate a potential raise of temperature from 1 °C (half of 2) to 4 °C (two times 2). This limits their usefulness but, at the moment, this remains the best tool we have.
Ovarian Scanning
Although the first described use of ultrasound in ob/gyn was for the diagnosis of an ovarian cyst [1], most research and publications have concentrated on obstetrics. This, however, changed with the recognition that ultrasound could be used to closely follow the ovarian cycle [45] and, subsequently, with the realization that this was an excellent tool in induction of ovulation and many other ART procedures [46–48]. Questions regarding safety of the procedure were raised immediately with the rapid adaptation by clinicians [2, 49–51], with description of premature ovulation [2], reduced fertility in rats [49], reduction in pregnancy rates in ultrasound-monitored groups [50], and lower fertilization rate in women undergoing artificial insemination and who were monitored by ultrasound as compared with those who were not monitored by ultrasound, and furthermore, those who were monitored took significantly longer to become pregnant [51]. No mechanism for the findings is proposed in any of these publications. Later, Doppler assessment of ovarian vasculature was also introduced [52–56]. Intraovarian vessels can be interrogated by color and spectral Doppler to predict ovarian response [57]. The acoustic outputs of these modalities are much higher than in conventional grayscale B-mode, but excellent, diagnostic images may be obtained with low outputs as documented by low TI and MI, as can be seen in Fig. 1.1. Further novel technologies to investigate ovarian vasculature, described in various chapters in this book, include three-dimensional (3D) ultrasound [33, 58, 59] and the use of contrast agents [60, 61]. While 3D ultrasound appears safe [34], the injection of contrast agents into the body greatly increases the risks of harmful bioeffects by introducing cavitation foci (see above). Ovarian scanning carries specific worries. In transabdominal scanning, a lot of energy is absorbed by the (sometimes thick) subcutaneous layers. In endovaginal scanning, this “safety net” does not exist since the probe is relatively close to the organ of interest, thus less absorption occurs. Higher frequencies of the vaginal probes, however, are protective. In addition, besides the direct effects of the ultrasound waveform, probe heating has to be considered. It is known that the surface of the probe can heat up by several degrees Celsius [62]. Most of this heat is dissipated by the abdominal wall tissues before reaching the ovary (or the fetus), but much less heat loss occurs with the endovaginal approach.
Ultrasound and the Ovum
As stated in the introduction, a study from 1982 demonstrated premature ovulation in women who underwent ultrasound examination of the ovaries (B-mode) in the late follicular phase [2]. The authors compared patients in induced ovulation cycles and investigated timing of follicle rupture after the onset of LH surge or administration of hCG. Rupture never occurred before the 37th hour in control patients (no ultrasound in the follicular phase). However, ovulation (premature) was observed at 26–36 h in about 50 % of cases in the study group (ultrasound during the previous 3 days or in the 36 h immediately following the ovulatory stimulus). This study was very concerning but has never been reproduced. Since its description 30 years ago [47, 63], ultrasound-guided oocyte aspiration for in vitro fertilization and embryo transfer has now become routine. There are only a few, relatively dated, studies aimed at determining the interaction between ultrasound exposure and successful fertilization. Most are, in fact, concerned with success or lack thereof of the procedure in terms of pregnancy rates and not possible bioeffects. This has not been studied with epidemiological methods but is, arguably, as important as analysis of embryonic/fetal effects. Some researchers have reported deleterious effects of ultrasound on the menstrual cycle, particularly decrease in ovulation rates in mice [64] and premature ovulation [2], as well as reduced cumulative pregnancy rates in mice [49] and in humans [50]. Others have demonstrated no effects on the ovulation process or egg quality, including DNA and RNA synthesis [65], nor on fertilization rate and embryonic development following in vitro fertilization and embryo transfer [66]. In general, the clinically available data on ultrasound exposure of oocytes during meiosis are confusing. Some researchers reported a deleterious effect on the fertility of patients undergoing artificial insemination with a reduction in the cumulative rate of pregnancy [50]. A study of ultrasound exposure of meiotically active, preovulatory oocytes showed no differences between rats exposed to ultrasound after the LH surge and controls in terms of pregnancy rate, number of corpora lutea, implantations, pups, and mean pup and placental weights at autopsy on day 22 of pregnancy [67]. Others have claimed an increase in the success rate, allowing ultrasound monitoring of follicular growth [68], although, evidently, this is not a direct effect of ultrasound but of improved timing. An attempt to clarify this was described by Mahadevan and colleagues [66]. They wanted to determine how oocytes obtained under ultrasound guidance affected the pregnancy rate. The results obtained with 3.5 MHz probes suggest that exposure of human oocytes to ultrasonic waves during the different phases of meiosis does not significantly influence the developmental potential of the in vitro fertilized embryos. Unfortunately, no researcher describes any of the relevant exposure parameters discussed earlier, except for ultrasound frequency.
Embryo/Fetus Susceptibility
The growing embryo/fetus is particularly sensitive to external influences. For instance, certain medications or drug of abuse taken by the pregnant woman, exposure to X-rays, and elevated temperature, secondary to infectious diseases, are all known teratological agents [69]. This is especially true in the first 10–12 weeks of gestation. Gestational age is thus a vital issue when dealing with possible bioeffects: milder exposure during the preimplantation period can have similar consequences to more severe exposures during embryonic and fetal development and can result in prenatal death and abortion or a wide range of structural and functional defects.
Several studies on the influence of ultrasound exposure in the preimplantation period are available. For instance, pregnant rats were exposed to a 2.5 MHz ultrasound field on the second and third day of gestation, at spatial average intensities of 150 mW/cm2, comparable to human exposure [70]. No increase in prenatal mortality was found. Similarly, no increase in the rate of postnatal malformation was found after 20 min exposures. In another experiment, pregnant mice were exposed to ultrasound in the first 3 days of gestation [71]. Spatial average intensity was determined to be 1 W/cm2. A decreased uninterrupted pregnancy rate was noted after exposure for 5 min on the third day and after exposure for 200 s on day 0. In addition, a reduction in neonatal weight (after delivery) was observed at certain thresholds for exposure on day 0 or 1. In another series of studies by the same authors [72], ultrasound exposure led to damage of maternal tissue, as reflected in increased mortality, decreased weight gain, and paralysis of the pups. One of the major concerns is whether ultrasound can raise the temperature of the developing embryo/fetus. This concern stems from the fact that, under certain conditions, ultrasound may indeed cause a rise of temperature and, on the other hand, it is well known that hyperthermia is teratogenic. Most at risk is the fetal central nervous system (CNS) due to a lack of compensatory growth of damaged neuroblasts [73]. In experimental animals the most common defects are of the neural tube as well as microphthalmia, cataract, and microencephaly, with associated functional and behavioral problems [74]. More subtle effects are possible, such as abnormal neuronal migration with unclear potential results [75]. Other prominent defects are seen in craniofacial development (more specifically facial clefts), the skeleton, the body wall, the teeth, and the heart [74]. Hyperthermia in utero (for instance, due to maternal influenza) has long been known to potentially induce structural anomalies in the fetus [76], but, relatively recently, it has been described as an environmental risk factor for psychological/behavioral disturbances [77] and, more particularly, schizophrenia [78]. It is stressed that these are not ultrasound-induced hyperthermia effects and that it is suggested that temperature elevation under 38.9 °C is probably not harmful. Yet, ultrasound has been shown to induce temperature increase in vivo [41], albeit not in humans. There is, however, a serious lack of data examining the effects of ultrasound while rigorously excluding other confounding factors. On the one hand, McClain and associates [79] exposed rats to 10 mW/cm2 CW Doppler ultrasound for up to 2 h at frequencies of 2.25 and 2.5 MHz. The fetuses were examined on day 20, and no consistent increase in mortality was observed nor did the authors detect any other abnormalities. Evidence, however, of the possibility of ultrasonically produced embryolethal effects during organogenesis has been described [80]. Sikov and colleagues exposed an exteriorized rat uterus to various frequencies, some of them clinically relevant (0.8, 2, and 3.2 MHz), at day 9 and evaluated the offspring at day 20 [81]. The exposure was performed at different intensity levels, with exposure times at 5 or 15 min. No effect on fetal weight was observed, even at spatial average intensities as high as 30 W/cm2, but prenatal mortality at 15–20 W/cm2 (spatial average) clearly increased with increasing exposure time. The cause of this was ascribed to a thermal mechanism. A recent controversial study looked at neuronal migration in rat pups after maternal exposure to ultrasound [75
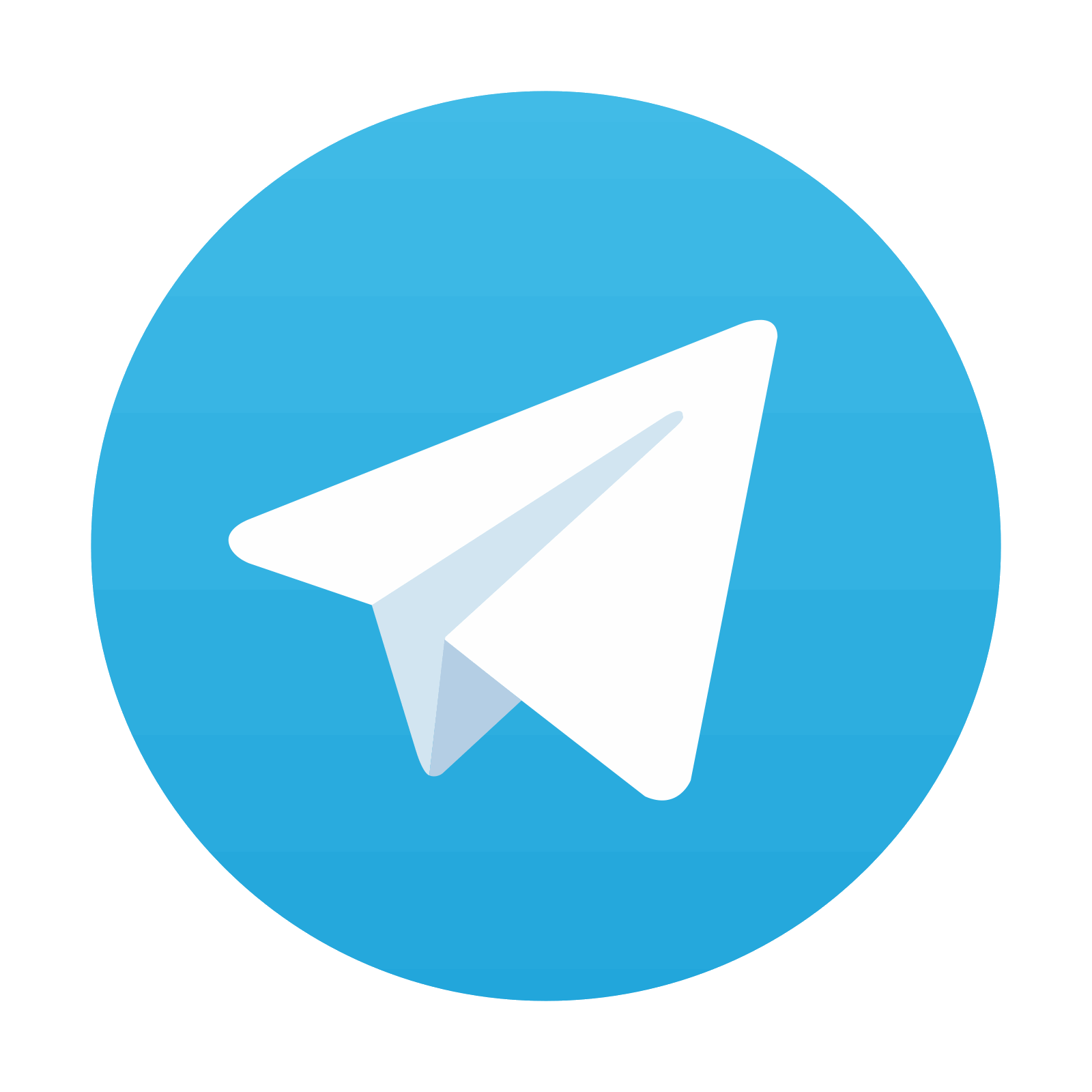
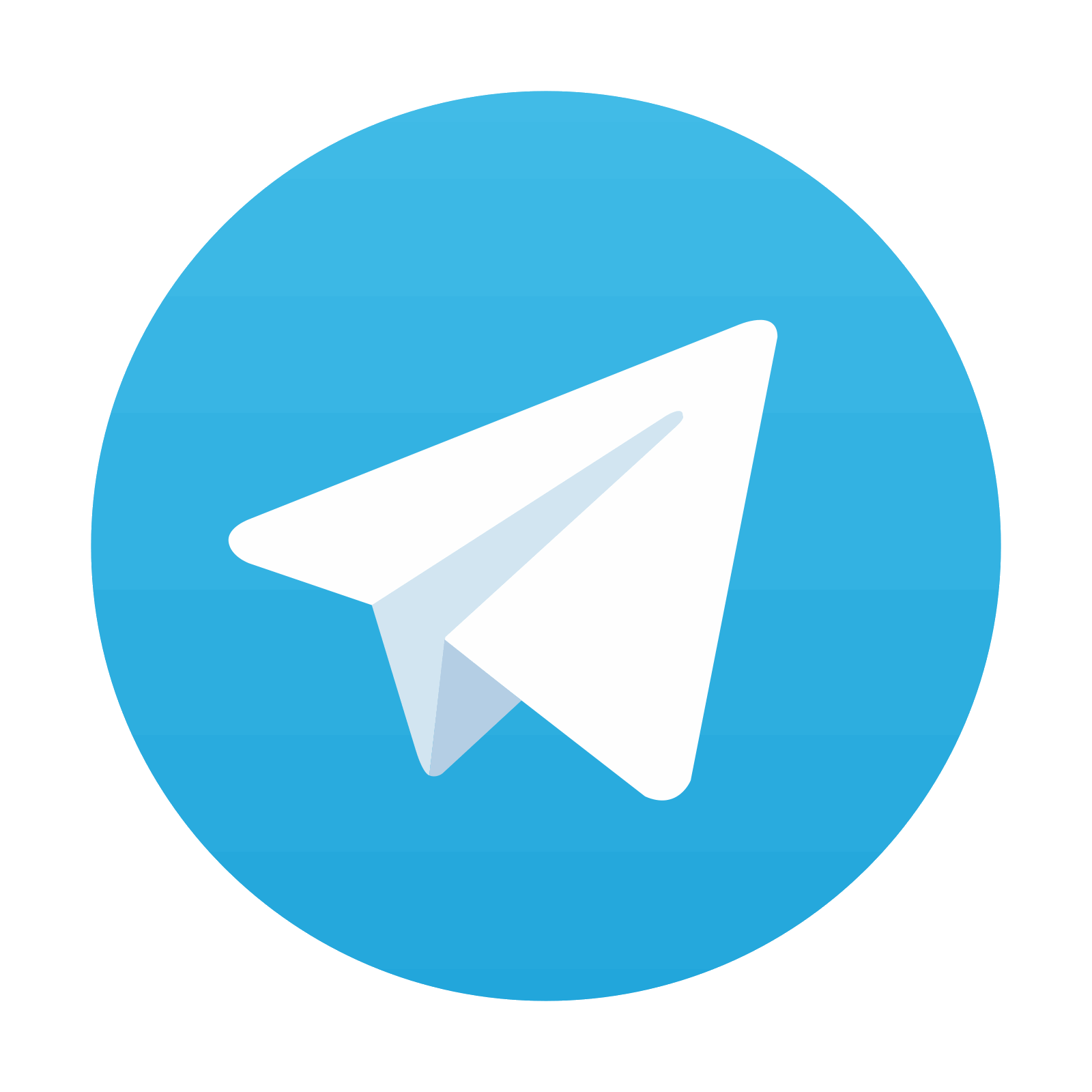
Stay updated, free articles. Join our Telegram channel
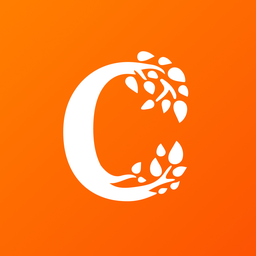
Full access? Get Clinical Tree
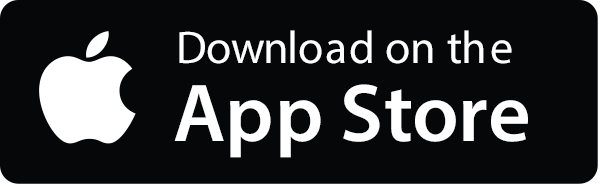
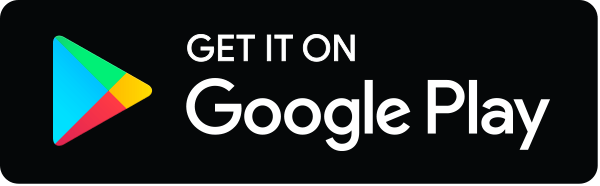