(6.1)
T represents the translatability of the technology in changing the existing clinical algorithm. A represents the magnitude of the application’s clinical impact, which considers individual impact, volume of patients affected, and societal impact. I represents the relative invasiveness of the application compared to alternative clinical strategies. S represents the rate of development and clinical translation of a technology. C represents the comfort level of practicing physicians in adopting the technology. Finally, R represents the relative resource impact perceived by decision-makers involved in implementing the technology. As such, a positive R represents resource gain while a negative value represents expenditure. Decision-makers generally include more than one entity, and the weighted interests and influence of the involved entities determine the value of the R term.
Using this formula helps explain why DTI has a high translatability. DTI has a high positive A value, or a superior clinical impact, because it is the most effective way to preoperatively visualize the relationships of white matter tracts to the resectable brain lesion. Details of this concept are covered later in the chapter. Because DTI is noninvasive, (A − I) is positive. Sequences are readily available on new MR systems and commercially available software makes workflow efficient and intuitive. Therefore, S is also positive. Although R may have a negative value in certain situations because of costs related to hardware or software upgrades to existing MRI scanners, this is easily overcome by the strongly positive clinical impact, A, and the increasing realization that DTI for presurgical white matter mapping is becoming the standard of care. Institutions without DTI capability will eventually be vulnerable to the perception of inferior clinical care and undesirable medicolegal scenarios.
The C value, comfort level of practicing physicians in adopting the technology, is critical. The practicing physicians are primarily the neuroradiologists and neurosurgeons. White matter functional anatomy is becoming an integral component of neuroradiology fellowship programs. Thus, for the newly trained neuroradiologist, C is actually positive. For the practicing neuroradiologist trained before the era of presurgical brain mapping, additional training in white matter functional anatomy is not insurmountable. In fact, the momentum from the high translatability of DTI will necessitate training in these individuals, propelling a mildly negative C value to neutral or positive. The C value as it relates to the neurosurgeon is perhaps even more integral to the translation of DTI. It is the neurosurgeon who directly puts this technology to use. Neurosurgeons who have recently trained at academic centers with clinical DTI and functional MRI (fMRI) demand this technology. They bear witness to the positive results of presurgical brain mapping and rely on it. The C value as it relates to the neurosurgeon is becoming increasingly positive. Neurosurgeons trained before the era of presurgical brain mapping may continue to perform tumor resections without this technology. However, it is at the patient’s disadvantage because DTI and presurgical brain mapping are the standard of care at many institutions.
Neurosurgery: Goals and Risks
The primary goals for neurosurgical resection of brain tumors are to establish a histological diagnosis and achieve maximal cyto-reduction. Because of the notorious histologic heterogeneity in gliomas, gross total resections are preferable to subtotal resections or biopsies for accurate diagnosis. With an accurate histologic diagnosis, the patient’s prognosis as well as the optimal treatment algorithm can be established. Treatment algorithms may include adjuvant radiotherapy and/or chemotherapy. Maximal cyto-reduction has been theorized to decrease cell populations that could convert to higher grades [1, 2] and improve the effectiveness of adjuvant therapies by altering cell kinetics and reducing cell populations resistant to chemoradiation. In point of fact, resection extent of high-grade and low-grade gliomas has been shown to correlate with survival [1, 3–6]. In terms of quality of life, up to 53 % of glioma patients may show improved neurologic function after resection [7]. Surgical excision of primary brain tumors can also decrease steroid dependence and seizure activity.
These benefits of histological characterization and cyto-reduction must be weighed against the risks. Brain tumor resections do not carry an insignificant risk to the patient. Prior to the era of modern presurgical brain mapping, neurological complication rates for brain tumor resections ranged from 7 to 26 % [7–15]. The most feared complications include both impairments to elementary functions such as motor and vision, as well as higher cognitive functions such as speech, language, and memory. DTI and presurgical brain mapping have had a profound impact on this risk–benefit analysis.
During initial internal translation of presurgical DTI at the Medical College of Wisconsin (MCW) in 2004, a pilot study included 33 left dominant high-risk posterior frontal lobe tumors that were resected [16]. Eighteen patients had tumors resected prior to DTI implementation while 15 had their tumor resections after preoperative DTI was available. New speech and motor deficits occurred in 44 % of the former group and 47 % of the latter group. However, as is often the case in the immediate postoperative setting, transient deficits were seen and there was varying recovery of these functions for both groups. In the group without presurgical DTI, 39 % of patients had persistent speech and motor deficits at 1 month after surgery. This contrasts with persistent speech and motor deficits at 1 month in only 7 % of the presurgical DTI patients. It follows that presurgical DTI resulted in significantly better recovery of neurological function (p < 0.05) following high-risk left frontal lobe tumor resections in age-, gender-, histology-, tumor size-, and location-matched controls performed by the same neurosurgeon with identical technique. The profound impact of DTI during this initial translation cemented its future at MCW for high-risk tumor cases with proximity to eloquent white matter structures. Since then, overall complication rates have fallen well below this 7 % benchmark [17].
Presurgical Mapping Process
Establishing an efficient workflow is necessary to create a reliable presurgical brain mapping service. Upon referral, usually by a neurosurgeon, neuropsychologist, or neurologist, the patient arrives for the DTI/fMRI exam. At this time, an outside MRI exam has already been loaded into the PACS or the patient hand carries a disc for the neuroradiologist to review. Upon review of the imaging, review of the electronic medical record, and direct patient interaction, the neuroradiologist selects specific mapping parameters including underlay anatomical sequences, the need for contrast, DTI protocol, and fMRI paradigms. The patient is then trained in the subselected fMRI paradigms, with any combination of language, motor, pre-motor, and vision categories. Patient experience for DTI is similar to conventional MR imaging and requires no special instructions. After the mapping data have been acquired, analyzed, and optimized for viewing, the neuroradiologist reviews the findings with the referring clinician(s). The mapping images are sent to the institutional PACS and then a decision is made whether or not to import the data into the intraoperative neuronavigation system. The presurgical mapping data then complement and guide intraoperative strategies, including white matter functional testing and electrocortical mapping.
Localization Sources
DTI is only one critical component of the presurgical and perioperative mapping process. There are five complementary preoperative and perioperative localization sources that establish functional network proximity risks necessary to preserve neurological function. These sources are clinical presentation, functional anatomy at standard imaging, presurgical functional mapping techniques (which include DTI), intraoperative functional white matter testing, and intraoperative electrocortical mapping. Each of these sources is imperfect alone, but can prove to be critical when combined with the others. Let us take a closer look.
Clinical presentation often yields valuable information regarding at-risk functional networks. Sources are available for detailed discussion of functional systems and lesion localization [18, 19]. The presenting neurologic deficit (sometimes associated with seizure) often indicates direct involvement or mass effect by tumor and/or edema upon a structure that is normally vital for carrying out that particular function. For example, a brain tumor patient presenting with a persistent or a transient seizure-induced language deficit indicates with a fairly high positive predictive value (PPV) that the lesion has proximity to eloquent structures. However, because of propagation of epileptogenic activity, the PPV is not 100 %. Assessments regarding the negative predictive value (NPV) in this capacity should not be made as seizures and deficits may be absent even though a lesion has proximity to eloquent structures.
Additionally, handedness determines the likelihood of left hemisphere language dominance [19, 20]. Right-handed individuals have a 98 % chance of being left hemisphere dominant for language function while left-handed individuals have a 67 % chance of being left dominant. The other 33 % of left-handers either are right hemisphere dominant for language or have shared hemisphere function. In the vast majority of patients, presenting neurologic deficit and handedness will accurately predict hemispheric language dominance. However, the importance of fMRI as a confirmatory test cannot be stressed enough. Consider that while 98 % of right-handed individuals are left hemisphere dominant for speech and language function, 2 % are not. That means for every 100 mapping cases performed on right-handers, two of them will have significant language function in the right hemisphere. Ignoring or not adequately scrutinizing language fMRI data could lead to significant postoperative speech and language impairment.
Knowledge of functional anatomy at standard imaging is critical in the interpretation of presurgical DTI data. In clinical practice, the color-coded FA maps are superimposed on anatomic imaging sequences to optimally characterize relationships of white matter structures to pathologic processes (Fig. 6.1a–c). For further discussion of color-coded FA maps see below. DTI by itself can be limited in establishing functional network proximity because of pathophysiologic constraints. For example, anatomic distortion of perilesional white matter from edema/infiltrating tumor may obscure and/or reorient tracts, making them difficult to discern at color-coded DTI (Fig. 6.2a, b). This is a frequent occurrence with high-grade gliomas. With the use of standard imaging, expected relationships of tracts to each other and to gyral and sulcal landmarks can help problem-solve and successfully predict functional network proximity when tract directions are altered or fractional anisotropy is nearly lost. In addition to aiding DTI interpretation, functional anatomy at standard imaging is key in the interpretation of fMRI, particularly in cases of decreased or absent cortical activation due to neurovascular uncoupling [21]. As mentioned previously, review of the pertinent functional anatomy on a patient’s standard diagnostic MR exam can aid in the selection of specific fMRI paradigms.
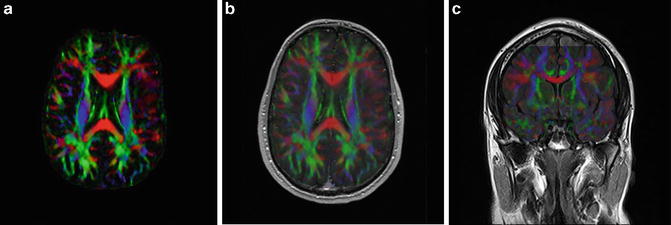
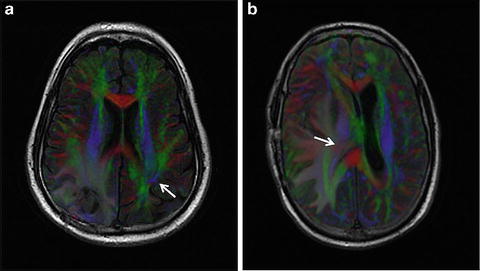
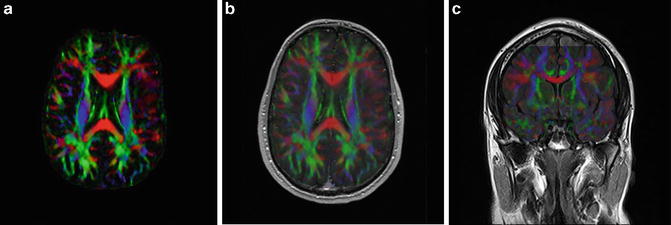
Fig. 6.1
(a) High-resolution axial color-coded FA map. (b) High-resolution axial color-coded FA map faded to 50 % and superimposed on an anatomic axial post-contrast SPGR image at the same slice location. (c) High-resolution coronal color-coded FA map superimposed on an anatomic coronal FLAIR image at the same slice location
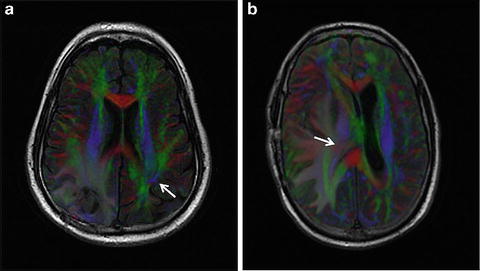
Fig. 6.2
Axial FLAIR images with superimposed, faded DTI data in two different patients with right-sided high-grade gliomas. In (a) perilesional tumor infiltration and/or edema results in markedly reduced white matter fractional aniosotropy. The right arcuate fasciculus cannot be confidently identified, unlike the preserved contralateral arcuate fasciculus (white arrow). In (b) a large right posterior temporal lobe lesion (not shown) creates marked mass effect on adjacent structures. Portions of the right corona radiata expected to contain corticospinal motor fibers are displaced transversely and appear red instead of blue (white arrow)
Presurgical mapping anatomy techniques include DTI, fMRI, magnetoencephalography (MEG), WADA testing, positron emission tomography (PET), MRI, MR spectroscopy, and computed tomography (CT). The goal of these techniques is to preoperatively define spatial relationships between a lesion’s borders and functional brain networks. At the heart of this lies the complementary duo of DTI and fMRI. DTI evaluates the white matter and fMRI evaluates the functional cortex. Value of DTI and fMRI data in a particular case usually depends upon the location of the lesion. Scenarios exist where cortical lesions have little proximity to functional white matter and deep lesions are remote to functional cortex. Most often, however, DTI and fMRI will provide useful and complementary information. Investigators found that the combined use of DTI, anatomic imaging, and fMRI was superior to anatomic imaging than fMRI alone for pre-operative functional system risk-proximity designations [22]. Optimal use of these techniques for presurgical brain mapping requires an understanding of the functional and dysfunctional anatomy. While there are good resources available for functional and dysfunctional anatomy reviews [18, 19], our understanding continues to evolve. Motor and vision functional networks are fairly well understood and have been deliberately avoided during neurosurgical procedures for many years. Primary motor cortex, SMA, corticospinal tracts, and corticobulbar tracts for motor function and visual cortex (especially occipital poles for central vision) and optic radiations (not Meyer’s loop) for visual function constitute identifiable structures at presurgical mapping. Conversely, identifying speech/language cortical regions and white matter tracts is complicated by the fact that our understanding of these functional networks is much less concrete. fMRI can give us indirect evidence of cortical language areas, though it is complicated by a combination of factors including temporal resolution limits, paradigm contrast, lesion-induced neurovascular uncoupling, and other artifacts. Autoradiographic tract tracing and DTI studies in nonhuman primates have revealed a tremendous amount of detail regarding white matter tract organization [23, 24]. Much of this information is presumed to be transferable to the human brain. Unfortunately, the lack of speech and language capabilities in nonhuman primates remains a major obstacle in applying these data to the human language system. Emerging theories of ventral and dorsal language streams [25–27] may aid in establishing which association tracts are necessary and thus to be avoided during surgery. At our institution, components of the dominant superior longitudinal fasciculus (SLF) including the arcuate fasciculus (AF) are considered eloquent structures and are intentionally preserved at the time of neurosurgical resections.
Intraoperative localization techniques include functional white matter testing and electrocortical mapping. When used in conjunction with lesion border-risk designations derived from presurgical mapping, these techniques can be utilized most efficiently. The neurosurgeon can tailor the use of these intraoperative localization techniques based upon the presurgical mapping data and thus reduce operation times. For example, the neurosurgeon can specifically test language and motor functions while dissecting along a lesion’s high-risk borders. Intraoperative electrical stimulation is one method used for functional white matter testing [28, 29]. At MCW, intraoperative functional dissection testing is performed. In conjunction with lesion border-risk designations derived from presurgical DTI, an ultrasonic aspirator is used that not only provides tissue fragmentation, irrigation, aspiration, and coagulation but also has a close-distance nonlethal and transient effect on neuronal tissue (Fig. 6.3a, b). If the patient develops a transient speech or language deficit upon dissection, impending functional white matter injury is predicted only millimeters beyond the dissection plane. A transient deficit limits resection along that tumor border. However, if a tumor border is deemed to be free of eloquent white matter at preoperative DTI, a grossly clean margin will be dissected using the ultrasonic aspirator. Intraoperative electrocortical mapping has its own advantages and disadvantages. This technique is not susceptible to the effects of neurovascular uncoupling and is especially valuable in cases where lack of cortical activation due to neurovascular uncoupling is suspected on presurgical fMRI [21, 30]. However, electrocortical mapping is limited in reaching eloquent cortex due to pial barriers and can be compromised by perilesional seizure induction. Electrocortical mapping and functional white matter testing are powerful tools, made more powerful by presurgical mapping data.
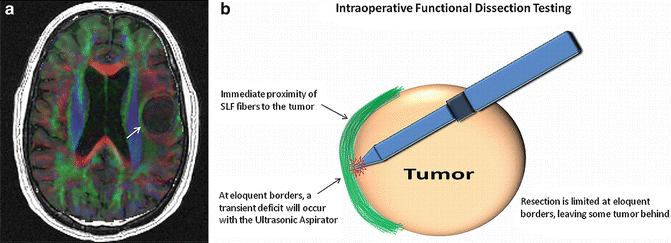
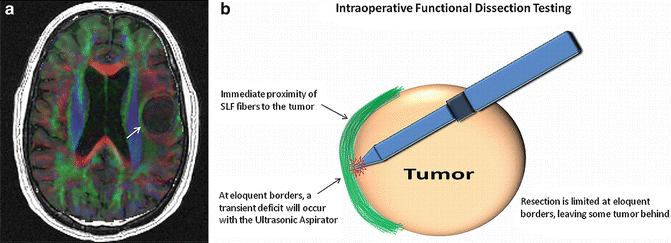
Fig. 6.3
In (a) there is a cystic lesion within the left frontal white matter. Horizontal fibers of the SLF (white arrow) are displaced and have immediate proximity to the medial border of the lesion. In a left hemisphere language dominant patient, this is considered a high-risk surgical resection. (b) illustrates the method of intraoperative functional dissection testing for this high-risk medial tumor border. Using lesion border-risk information derived from presurgical DTI, language function is tested while the neurosurgeon dissects with the ultrasonic aspirator. If a transient language deficit occurs, an impending permanent deficit is likely if dissection is continued along that plane. Therefore, functional white fibers along this border are spared and some tumor is left behind
DTI Protocols, Acquisition
For CC-FA maps, a dual-refocused spin echo technique is used to minimize distortions and maximize signal-to-noise ratio (SNR). DTI resolution is determined by readout time and gradient strength. Diffusion weighting and higher resolution reduce SNR. Greater diffusion gradient encoding directions lengthen the duration of the DTI scan. At least 6 orthogonal gradient encoding directions are required to construct a diffusion ellipsoid, but 12 or more are preferred to minimize directional under-sampling at fiber crossings and at acute angulations. However, some venders only allow odd numbers of encoding directions for reasons of colinearity. The CC-FA sequence protocols at MCW were designed by Wolfgang Gaggl in his capacity as research engineer. At 1.5T, 3 separate data sets with 3 mm slice thickness, 13 gradient encoding directions, b value of 900, NEX of 2, TE of 70 ms, and TR min of 11 s are used to acquire a total of 40 contiguous slices. Typically, 128 × 128 matrix and an FOV = 20−24 cm will be acquired, with in-plane resolution generally at 1.8 × 1.8 mm. Some vendors will automatically interpolate the DTI data to 256 × 256, which has a smoothing effect. Acquisition time is 5.5 min for each data set, with a total time of 16.5 min for all three data sets. The Gaggl modular approach provides the latitude to modify the slice thickness and gain necessary for adequate SNR simply by changing the number of runs. Three averaged data sets at 1.5T provide an SNR equivalent to a single run with an NEX = 6, with SNR to spare. If a patient can only withstand one or two 5.5-min acquisitions, the data are aesthetically compromised, but still diagnostic. For 2.5 and 2 mm slice thicknesses, however, four and six averaged 5.5-min data sets, respectively, are required to achieve diagnostic SNR. Movement between data acquisitions can be corrected in post-processing. The DTI data are suitable, though not optimal, for fiber tracking if needed (see fiber tracking section below).
At 3T MRI sequence parameters are similar, though slice thickness is typically reduced, higher TR may be needed, b value = 1,000 or greater, and up to 50 slices are acquired. For 3 mm slice thickness, two data sets are averaged, but we generally acquire data at 2 mm slice thickness requiring three acquisitions to achieve nearly isotropic voxels of 1.87 × 1.87 × 2 mm with sufficient signal. DTI data at 1.5T or 3T are acquired in the axial plane and reconstructed in coronal and sagittal planes and superimposed onto desired underlay images for all cases. Because DTI is acquired with other mapping data, including fMRI, patients with prior surgeries or biopsies are scanned at 1.5T to reduce susceptibility. Sequence parameters are customized to optimize patient compliance and minimize motion effects, depending on the length of time required to acquire DTI and other mapping data. Generally though, three runs at 3 mm (1.5T) or 2 mm (3T) slice thicknesses suffice.
DTI Data Visualization
DTI is rooted in the concept that white matter microstructure, primarily axons, provides a barrier for random diffusion of water molecules. By acquiring data along at least six different gradient directions, ellipsoids representing relative directional movements of water can be calculated. Each ellipsoid is defined by one major and two minor eigenvectors based on the major and minor directions of water movement. The ellipsoid, or diffusion tensor, is the basis by which relative directional information of white matter can be displayed on a voxel-by-voxel basis.
Information from the three components of the diffusion tensor vector can be displayed in color-coded FA maps. These maps enable one to visualize the anatomic organization of white matter tracts with the added value of directionality. At any one voxel on the image, one of the three colors is assigned according to the eigenvector with the greatest eigenvalue. By convention, green represents the anteroposterior (or posteroanterior) direction, red the transverse (right to left or left to right) direction, and blue the craniocaudal (or caudocranial) direction [31]. Color intensities are scaled in relation to the magnitude of the FA value. A benefit of color-coded FA maps is the ability to visualize global white matter architecture by the same method conventional MR images are displayed, i.e., in the axial, coronal, and sagittal planes. With a thorough understanding of white matter anatomy, a tremendous amount of valuable information can be garnered prior to surgery. DTI atlases are available for a detailed illustration of white matter anatomy [32]. At our institution, the color-coded FA map is the primary method by which DTI data are utilized in the presurgical mapping process.
Fiber tracking, or tractography, is an additional means of displaying DTI data. A three-dimensional representation of a white matter tract is constructed based on the directionality information derived from FA values. Using mathematical algorithms, fiber tract trajectories are estimated based on the major eigenvector at sampled locations, propagating from a “seed point.” Numerous different DTI tractography algorithms have been proposed in the literature, either deterministic or probabilistic [33–37]. The former method only uses the best estimate of the major eigenvector to propagate the fiber trajectories, while the latter includes a method for estimating and displaying the uncertainty in propagation between two points. Most commercially available fiber tracking software packages coupled with scanners have a deterministic mathematical algorithm.
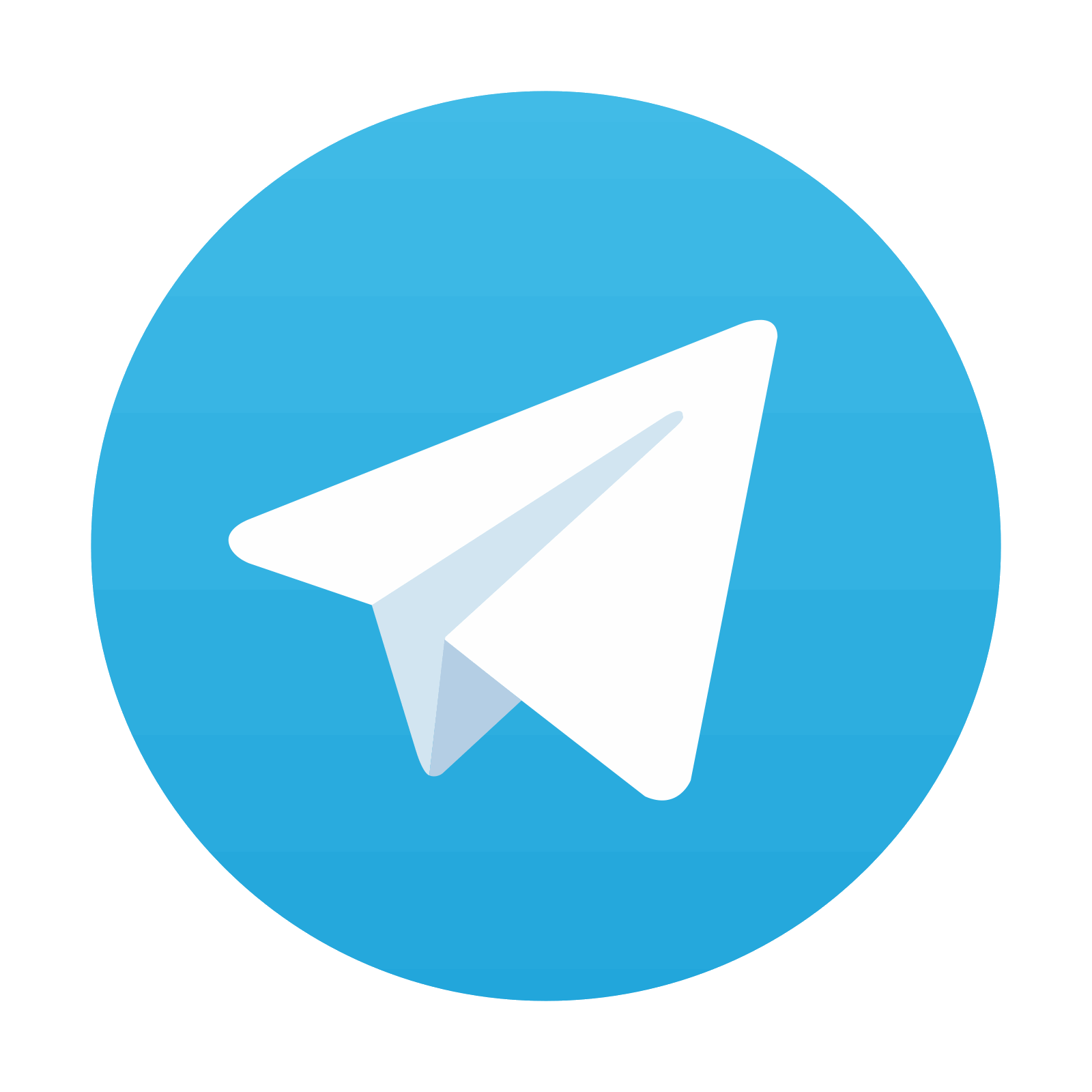
Stay updated, free articles. Join our Telegram channel
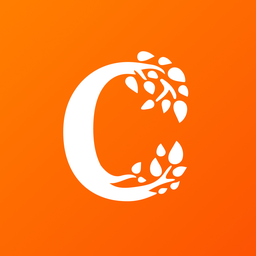
Full access? Get Clinical Tree
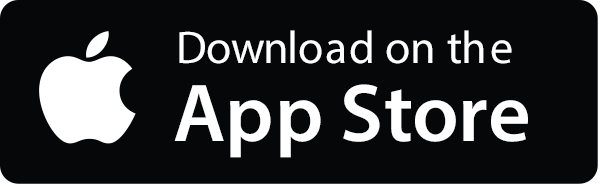
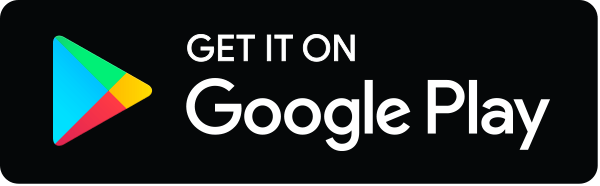