Fig. 1
Mechanism of RNA interference. Long dsRNA introduced into the cytoplasm is processed by the enzyme Dicer into 22-nt pieces with 2-nt single-stranded overhangs on the 3′ ends. The structure of synthetic siRNA mimics that of Dicer products. The siRNA guide strand is loaded into the RNA-induced silencing complex (RISC), and the passenger strand is cleaved by Argonaute-2 (Ago2). The activated RISC–guide-strand complex identifies and cleaves mRNA that is complementary to the guide strand, preventing translation and thereby silencing gene expression. Reproduced with permission from Ref. [4]
Despite its promise, siRNA has not realized its potential as a therapeutic because of obstacles related to its delivery in vivo. After intravascular administration, siRNA is susceptible to degradation by RNase A-type nucleases or is aggregated by serum proteins in the plasma, and is rapidly eliminated by the kidneys, resulting in very short intravascular circulating half-lives in the range of seconds to minutes [5]. After entering the circulation, siRNA has to pass across vascular endothelial walls and diffuse through the extracellular matrix (ECM) in the interstitium. This represents a significant delivery hurdle since the ECM forms a dense network structure consisting of polysaccharides and fibrous proteins that accommodate macrophages. Finally, siRNA delivery is limited by the negatively charged membranes of target cells mainly because the negative charges on the phosphodiester backbone and the large molecular weight of siRNA (~13 kDa) hinder uptake by mammalian cells. In addition, siRNA could induce an innate immune response that is mediated by type I interferon and proinflammatory cytokines , further limiting the feasibility of delivering unmodified naked siRNA in vivo [6, 7].
To overcome these obstacles, a wide range of nano-sized delivery vehicles (nanodrugs) has been investigated. The nano-delivery agents can be classified into three types: lipid-based, polymer-based, and inorganic nanodrugs. Considering the obstacles listed above, an optimal nanodrug should be equipped with the physicochemical properties of protecting siRNA from degradation, elongating blood circulation, localizing in desired loci in the body, facilitating the cellular uptake, and releasing siRNA within the cytosol to initiate the RNAi process. Also, nanodrugs are expected to satisfy requirements related to their physiological safety, including lack of immunogenicity, noncoagulation with serum proteins, and low nonspecific uptake by normal tissues or cells.
To accommodate the requirements for efficient siRNA delivery, nanodrugs are mainly optimized in terms of surface charge and particle size. The outer surface of nanodrugs is usually charged with cations (positive charges) for enhanced uptake by cell membranes. However, cationic surface charges increase the chances of aggregate formation of negatively charged plasma proteins that are entrapped in the pulmonary capillary bed or taken up by the mononuclear phagocyte system. The introduction of polyethylene glycol (PEG) on the surface can neutralize the surface charge and impart a “stealth function” that avoids the interactions with plasma proteins, resulting in the elongation of intravascular circulation time and faster diffusion in the extracellular matrix [8]. In contrast, PEGylation interferes with spontaneous cellular uptake through opsonization and requires an additional moiety for receptor-mediated endocytosis. With respect to nanoparticle size, nanodrugs are limited by the requirement that the size of nanodrugs should be bigger than the pore size of the glomerular filtering system (>7 nm) to avoid renal clearance. It is also beneficial to keep the size smaller than 100 nm because the discontinuous endothelia in solid tumors are also found in some other organs including liver, spleen, and bone marrow, where nanoparticles bigger than 100 nm in diameter are entrapped [9–12].
The conventional size of nanodrugs is adjusted in the range of 10–100 nm in diameter to allow delivery to tissues through the enhanced permeation and retention (EPR) effect. This is particularly relevant for solid tumors with leaky vasculature [9, 13, 14]. Even in poorly permeable tumors, nanodrugs smaller than 50 nm can penetrate the capillary endothelium, whereas micellar nanodrugs of 70 nm are retained in the vasculature [15]. Based on these observations, the optimal size of nanodrugs can be narrowed to 10–50 nm, especially when the goal is passive delivery for cancer treatment.
In this review, we will focus on the formulation of nanodrugs for pharmacological siRNA delivery according to the categories classified above: lipid-based, polymer-based, and inorganic nanodrugs.
2 Polymeric Nanoparticles
Polymer-based nanoparticles have numerous advantages as a template for siRNA nanodrugs including thermodynamic stability, high drug loading efficiency, high cellular uptake, adjustable drug release rate, and the feasibility of surface modification. However, polymeric nanodrugs have potential issues originating from the properties of synthetic or natural polymers, such as biodegradability, biocompatibility, toxicity, and immunogenicity.
Polyethyleneimine (PEI) is one of the most commonly used polymers. PEI-based nanoparticles show high cellular uptake due to their cationic surface charges. PEI exhibits very different compatibility profiles depending on its incorporation of specific molecular structures and the choice of branched (obtained from acid-catalyzed polymerization of aziridine) vs. linear forms (obtained via polyisoxazoline precursors) [16, 17]. With an acceptable biocompatibility profile, nanodrugs formulated with linear PEI show no significant increase of proinflammatory cytokines or hepatic enzymes after systemic administration [18, 19]. Branched PEI is recognized as more toxic than linear PEI, activating inflammatory responses and susceptible to macrophage uptake and rapid clearance. Chemical modification can improve the toxicity profile of branched PEI. PEGylation of branched PEI prevented the induction of mutations and oxidative DNA damage (8-OH-dG) in FE1 lung epithelial cells, whereas alkylation showed low toxicity and enhanced knockdown efficiency due to stabilization of encapsulated siRNA [16, 20, 21].
In addition to PEGylation and alkylation , PEI has been complexed with lipids, polymers, surfactants, and inorganic materials to enhance the properties of nanodrugs [22–27]. For example, low-molecular-weight polyamine (PEI600) was utilized for the synthesis of nanoparticles by reacting with C15 epoxide-terminated lipids at a 14:1 molar ratio, and formulating the nanoparticles with C14PEG2000. The resulting nanodrugs formed multilamellar vesicles rather than the periodic aqueous compartments containing siRNA that make up stable nucleic-acid lipid particle formulations. The resulting nanodrugs had a diameter between 35 and 60 nm, and were delivered into endothelial cells without any target moieties. The surface charge of 7C1 nanodrugs was electrically neutral at pH 7.4 (in the blood stream), but its pK a was 5.0. Unlike lipid and lipidoid-based nanodrugs, 7C1 nanodrugs showed high specificity of transfection to endothelial cells in vivo even at low doses, and did not significantly reduce gene expression in nontargeted cells, such as hepatocytes, peritoneal immune cells, pulmonary epithelial cells, or pulmonary immune cells (Fig. 2) [27].
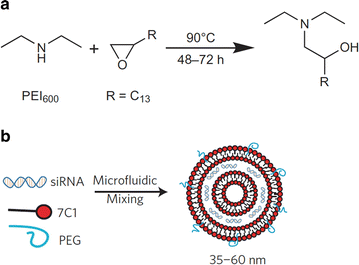
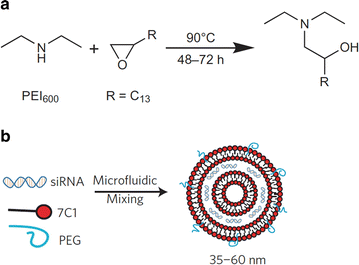
Fig. 2
Preparation of polymeric nanoparticles with low molecular weight. (a) 7C1 synthesis scheme. (b) 7C1 formulation scheme. 7C1 nanoparticles were mixed with C14PEG2000 and siRNA in a high-throughput microfluidic chamber. Reproduced with permission from Ref. [27]
Co-delivery of two siRNAs and paclitaxel was investigated by employing a two-amphiphilic polymer system of polyethyleneimine-block-poly[(1,4-butanediol)-diacrylate-β-5-hydroxyamylamine] (PEI-PDHA) and polyehtyleneglycol-block-poly[(1,4-butanediol)-diacrylate-β-5-hydroxyamylamine] (PEG-PDHA) by self-assembly. PDHA is acid responsive and releases drugs in an acidic environment. Two siRNAs and paclitaxel were encapsulated in polymeric particles to form PEI-PDHA/PEG-PDHA/PTX/siSna/siTwi (PPSTs) complex nanodrugs. The size of PPSTs nanodrugs (80–140 nm) was small enough to expect passive targeting through EPR effects. PPSTs nanodrugs were administered intravenously, and the accumulation of two siRNAs and paclitaxel was confirmed in metastatic 4 T1 breast tumors in a mouse model. The administration of dual siRNAs resulted in suppression of breast cancer by preventing cancer cell migration and invasion and the effect was more significant than the administration of single siRNA [28].
An abundance of polymers has been explored for the delivery of anticancer therapeutics, Some examples include poly(lactide-co-glycolide) (PLGA) , polylactide (PLA) , polyglycolide, polycaprolactone (PCL) , and poly(D,L-lactide). These polymers have also been chemically modified with lipids, other polymers, surfactants, and inorganic materials to improve the physicochemical properties and optimize the formulations for siRNA delivery [29–34]. For the treatment of bladder cancer , for example, PLGA nanoparticles were modified with positively charged mucoadhesive polysaccharide chitosan chains (2.5 or 20 kDa) to enhance transurothelial penetration and delivery of survivin siRNA. Surface modification of PLGA nanoparticles with short chain chitosan (NP-siSUR-2.5, 137 ± 51 nm) increased the release of siRNA by ten times compared to long chain chitosan (NP-siSUR-20, 130 ± 56 nm). Nanodrugs were delivered using passive targeting, since no targeting moieties were incorporated into the design. The higher molecular weight (long chain) chitosan entangled and trapped the negatively charged siRNA more tightly than the lower molecular weight (short chain) chitosan [34, 35]. This result implies that the balanced surface charge should be optimized for enhanced cellular uptake and siRNA release into the cytosol.
The formulation of nanoparticles using natural polymers has also been explored for the delivery of siRNAs. Representative natural polymers are chitosan, hyaluronic acids, sodium alginate, gelatin, and polypeptides [36–41]. Glycol chitosan was chemically modified with 5β-cholanic acid to form a hydrophobic core where doxorubicin (Dox) was encapsulated in self-assembling nanoparticles. Also, siRNA was conjugated to a thiolated glycol chitosan via a cleavable disulfide for release in the reductive intracellular environment. Interestingly, Dox-encapsulated CNPs (290 ± 4.5 nm) or Bcl-2 siRNA-encapsulated CNPs (301 ± 9.3 nm) exhibited similar physicochemical properties, including size, surface properties, and pH sensitive behavior, regardless of the different physical features of Dox and Bcl-2 siRNA . Also, the two different nanodrugs showed similar patterns of in vivo biodistribution and pharmacokinetics in PC3 tumor bearing mice, and mediated a dose-dependent therapeutic effect following sequential administration [41].
Dendrimers represent another common formulation for siRNA delivery. The most widely used dendrimers consist of polyamidoamine (PAMAM, Starburst™), poly(propylenemine) (PPI or DAB, Astramol™), and PEG polyester dendrons, and are functionalized with a –NH2 (for oligonucleotide delivery), a –COOH (for dendrimer platinates), and an –OH (for dendrimer-derived magnetic resonance imaging contrast agents). The size of dendrimers can be regulated by stepwise growth and adjustable branching patterns. Their hydrodynamic diameter is well defined according to the degree of generations, such as generation 3 (G3, 3.1 nm), generation 4 (G4, 4.0 nm), and generation 5 (G5, 5.3 nm). The surface functional groups allow for the adjustment of surface charges and permit functionalization with siRNA, targeting probes and surface coating materials [42–47]. However, dendrimers, especially cationic dendrimers, suffer from low biocompatibility and toxicity limiting their clinical translation [48, 49].
Dendrimer-inspired nanodrugs were developed by combining PAMAM or poly(propylenimine) dendrimers of increasing generations with alkyl epoxides of various carbon chain lengths. In this scenario, the positively charged dendrimer core entrapped negatively charged siRNAs for Tie2 gene knockdown in immortal and primary endothelial cells. 1,2-dimyristoyl-sn-glycero-3-phosphoethanolamine-N-mPEG2000 and cholesterol were incorporated with the dendrimers to generate nanodrugs with sizes in the range of 50–100 nm. Contrary to low molecular weight PEI-based polymer that was capable of targeting both lung endothelial cells and epithelial lung tumors, this formulation of Tie2 siRNA nanodrugs was specifically taken up by lung endothelial cells and not by epithelial lung tumor cells, whereas the integration of cholesterol improved gene knockdown efficiency. However, this formulation was not completely free from off-target effects in the endothelial cells of other organs because some knockdown was still observed at high doses [47].
3 Lipid-Based Nanoparticles
Lipid based nanodrugs include liposomes, solid lipid nanoparticles, stable nucleic acid-lipid nanoparticles (SNALPs) , and lipoid nanoparticles. Liposomes are the most popular and extensively investigated lipid-based nanoparticles and are employed to deliver a variety of payloads, such as anti-cancer drugs, oligonucleotides, DNAs, RNAs, antigens, and proteins. The advantages of liposomes for siRNA delivery include the prevention of degradation, accumulation in tumor tissues by passive targeting, feasibility in surface modification for active targeting, and high biocompatibility for systemic delivery in animals and humans. Described in “polymeric nanodrugs ,” liposomes are coated with polyethylene glycol (PEG) to avoid rapid clearance by the reticuloendothelial system (RES) in liver, spleen, lungs, and bone marrow for a longer circulation half-life. Also, liposomes are formulated with polymers, lipidoids, inorganic materials, and polypeptides to improve physicochemical properties for efficient siRNA delivery [50–55].
Layer-by-layer (LbL) nanoparticles are prepared by sequential deposition of oppositely charged polymers on top of nanoparticles to build a highly stable multilayer film. This permits precise control at the nanometer-scale level to adapt a range of polycationic materials with siRNA loading and releasing, film stability, transfection efficiency, and cytotoxicity. LbL nanodrugs were developed for co-delivery of MRP1 siRNA and anticancer therapeutics by stacking siRNA-loaded LbL films atop of a doxorubicin-loaded liposome with an exterior negatively charged phospholipid membrane. Cationic poly-l-arginine was deposited in the inner most layer to reverse the surface charge of the liposome from negative to positive which can drive negative siRNA molecules to form an outer shell in the LbL film layer. The size of the nanodrugs was determined to be approximately 120 nm in diameter with a zeta potential of 55 mV and the hydrodynamic diameter increased by 5 nm with the addition of each layer. The number of siRNA molecules per nanoparticle was determined to be 3500. The LbL nanodrugs were finalized with a hyaluronic acid (2000 kDa) coat for prolonged circulations with a serum half-life of up to 28 h [55].
In addition to liposomes, solid lipid-based nanodrugs were also developed for systemic siRNA delivery with the benefit of using biodegradable and nontoxic lipids that form solid nanoparticles at a physiological body temperature. These nanodrugs include “stable nucleic acid-lipid particles (SNALPs) ” and “solid-lipid nanoparticles (SLNs) .” SNALPs consist of a lipid bilayer containing a mixture of cationic and fusogenic lipids that enable the cellular uptake and endosomal release of siRNA. SLNs are composed of cholesteryl ester, triglyceride, cholesterol, dioleyl phosphatidyl ethanolamine (DOPE), and 3-β-[N-(N′,N′-dimethylamino ethane)carbamoyl]-cholesterol (DC-cholesterol). The formulations of SNALPs and SLNs are also modified with PEG groups, lipids, surfactants, polymers, and human serum proteins, and are adjusted for the purpose of siRNA delivery [53, 56–62].
Lipidoid nanoparticles are lipid-like delivery molecules conjugated with cholesterol and formulated with PEG-coated lipids for delivery of siRNAs [63–66]. For the delivery of osteogenic Plekho1 siRNA, lipid nanoparticles (size: <90 nm) were formulated to encapsulate siRNAs by use of DPPC, C16 celarmide-PEG2000, and cholesterol, and modified with the osteoblast-specific aptamer CH6 on the surface. The siRNA encapsulating lipid nanoparticles showed in vitro osteoblast selective uptake via macropinocytosis, and down-regulated in vivo osteoblast-specific Plekho1 gene expression, which resulted in promoted bone formation, improved bone microstructure, increased bone mass, and enhanced mechanical properties in both osteopenic and healthy rodents (Fig. 3) [62].
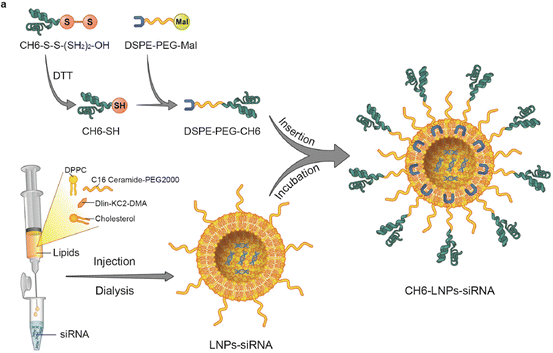
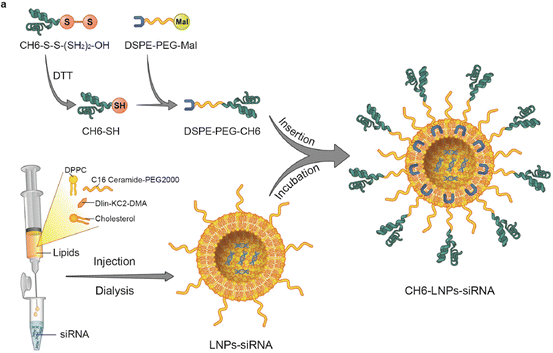
Fig. 3
Preparation of aptamer-functionalized lipid nanoparticles. LNPs are first prepared by spontaneous vesicle formation after a lipid/ethanol solution is slowly injected into siRNA buffer solution, followed by dialysis. The 3′ thiol and 2′-O-methyl-modified CH6 aptamer are then activated followed by conjugation to DSPE-PEG2000-Mal to form CH6-PEG2000-DSPE. Finally, CH6-PEG2000-DSPE in the form of micelles is inserted into the surface of LNPs. Reproduced with permission from Ref. [62]
4 Inorganic Nanoparticles
Inorganic nanoparticles can be classified as metallic nanoparticles and nonmetallic nanoparticles. Metallic (or metal-hybridized) nanoparticles include gold [67–70], silver [71], copper [72], manganese oxide [73], and iron oxide [74, 75], as well as quantum dots [76–78]. Nonmetallic nanoparticles include silica (silicate) and calcium phosphate (hydroxyapatite) [79–84]. These inorganic nanoparticles can be modified with PEG groups, polymers, lipids, and surfactants for the enhancement of drug loading, blood circulation time, and specificity to the target biomarkers.
Magnetic nanoparticles (MNs) have been used for the purpose of contrast enhancement in magnetic resonance imaging (MRI) and have also been employed for the delivery of siRNAs. In terms of diagnostic capability and delivery of siRNA therapeutics, MNs are considered a principle template for theranostic imaging. For the investigation of drug resistance in glioma against DNA-methylating agents, amine-derivatized dextran coated MN was loaded with siRNA for silencing O6-methylguanine methyltransferase (MGMT) , which reverses the anti-glioma effects of DNA methylating anticancer drugs. The MNs were also conjugated with a chloride ion specific peptide, Chlorotoxin , for effective uptake by glioma cells. By suppressing MGMT gene expression in an intracranial glioma model, the therapeutic effects of Temozolomide (DNA-alkylating anti-glioma agent) were significantly increased (Fig. 4) [74].
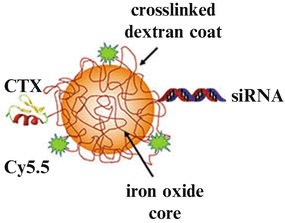
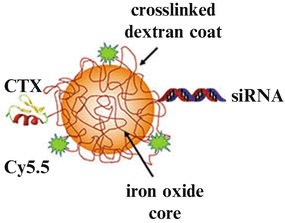
Fig. 4
Schematic structure of a nanodrug for targeted delivery of siRNA. Reproduced with permission from Ref. [74]
Nanodrugs loaded with siRNA are taken up by cells through endocytic pathways, and are prone to entrapment within subcellular compartments, which requires an increased dosage of siRNA. Polycationic nanodrugs can enhance the cellular uptake and facilitate the escape of siRNA from endosomes by taking advantage of the “proton sponge effect” [85], but can also cause cytotoxicity. For the optimal delivery of siRNA into the cytosol, nanoparticle-stabilized nanocapsules (NPSCs) were designed based on supramolecular guanidine–carboxylate interactions between the arginine-functionalized gold nanoparticles (Arg-AuNPs) of the shell and the hydrophobic fatty acid “oil” components in the core. NPSCs were successfully shown to be effective siRNA delivery vehicles into the cytosol by their ability to silence green fluorescent protein (deGFP) and polo-like kinase (siPLK1) with concomitant cytotoxicity [86].
Mature dendritic cells (DCs) can capture tumor antigens and induce potent antigen-specific antitumor immunity in tumor-draining lymph nodes by presenting the antigens to T cells and secreting proinflammatory cytokines that enhance T cell activation. Activation of STAT3 in DCs inhibits the expression of numerous immunostimulatory molecules triggered by TLR ligands that regulate T cell activation. For the suppression of STAT3, amine-modified quantum dots were conjugated with STAT3 siRNAs and encapsulated in a PLGA matrix. After intratumoral injection, STAT3 mRNA expression was downregulated by up to 73 % and the amount of proinflammatory cytokines increased significantly [87].
Porous silicon nanoparticles (pSiNPs) are actively investigated as nanoparticle-based drug delivery vehicles and have advantages in terms of biocompatibility, biodegradability, and high payload capacity [88, 89]. The pore sizes of pSiNPs can be controlled and provide criteria to classify SiNPs, such as micropores (smaller than 2 nm) through mesopores (between 2 and 50 nm), and macropores (larger than 50 nm) [90]. pSiNPs loaded with MRP1-siRNA showed efficient cellular uptake by T98G glioblastoma cells (even after a 30 min incubation) and successfully downregulated MRP1 mRNA (40 %) and protein (30 %) [91].
The delivery efficiency of silicon particles inside the body is affected by the size, shape, and surface physical and chemical properties of the nanoparticles. Discoidal silicon particles are more effectively taken up by tumor cells than spherical or cylindrical silicon particles. In addition, systemic administration of the discoidal SiNPs does not cause acute or subacute toxicity in wild-type mice [92, 93]. Polycation-functionalized porous silicon (PCPS) was prepared for the delivery siRNA. PCPS was fabricated by oxidizing the surface of the porous silicon to introduce a hydroxyl group that was used to conjugate arginine and PEI through a linker (3-aminopropyl-triethoxysilane). Loading of STAT3 siRNA was achieved through electrostatic interaction between the positively charged Arg-PEI on PCPS and the negatively charged siRNA. After uptake by breast tumor cells, STAT3 siRNA was released in the process of PCPS degradation to induce a significant 91 % knockdown of the target gene [94].
5 Future Outlook
RNA interference (RNAi) holds great potential as a therapeutic strategy for the treatment of many diseases, including cancers. RNA interference can be accomplished by two routes: the “chop-up” or “zip-up” route. In the zip-up mechanism, unnatural synthetic RNA oligonucleotides are employed to form a thermodynamically and physicochemically stable complex with target RNAs, which are not able to participate in the gene translation process. Following the chop-up mechanism, siRNAs are synthesized by the use of natural RNA building blocks and are utilized for the interference of target gene expression by binding the target gene to form a partial duplex in the sequence that is prone to RISC digestion. Therefore, siRNAs consist of natural RNA bases and are easily degraded by nucleases in sera. The role of nanoparticles is to protect siRNAs during circulation in blood vessels and diffusion in interstitium, to safely deliver siRNAs to the desired loci, and to release siRNA for silencing target mRNAs. So far, a wide range of organic, inorganic, and hybrid materials have been investigated for the purpose of siRNA delivery to the desired loci in vivo. The key challenge, however, is to translate the application of pharmacologic siRNAs into the clinic. This capability can have a transformative impact on healthcare because siRNAs provide incomparable specificity, including the capability to target single-nucleotide mutations.
In addition to their high level of specificity, siRNAs also have a broad applicability. Theoretically, we can target any genes using a modular strategy. This is important because practically every disease is influenced by genetic predisposition, including diseases that are caused directly by environmental factors. So far, a very limited number of therapeutic siRNAs have been evaluated in clinical trials for the treatment of diverse diseases, such as diabetic macular edema, immunotherapy of melanoma, myeloid leukemia, metastatic solid tumors, and liver cancer. Twenty clinical studies have been completed already and eight clinical studies are planned for the evaluation of siRNA in patients (clinicaltrials.gov). Considering the increasing number of preclinical investigations, it is reasonable to expect that the near future will see many more siRNA-based pharmacologic agents tested as monotherapies or combination therapies. However, before siRNAs can fully realize their potential in a clinical setting, it is imperative to develop optimized nanodrug platforms that are both safe and efficient.
References
1.
2.
3.
McCaffrey AP, Meuse L, Pham T-TT, Conklin DS, Hannon GJ, Kay MA. Gene expression: RNA interference in adult mice. Nature. 2002;418(6893):38–9.CrossRefPubMed
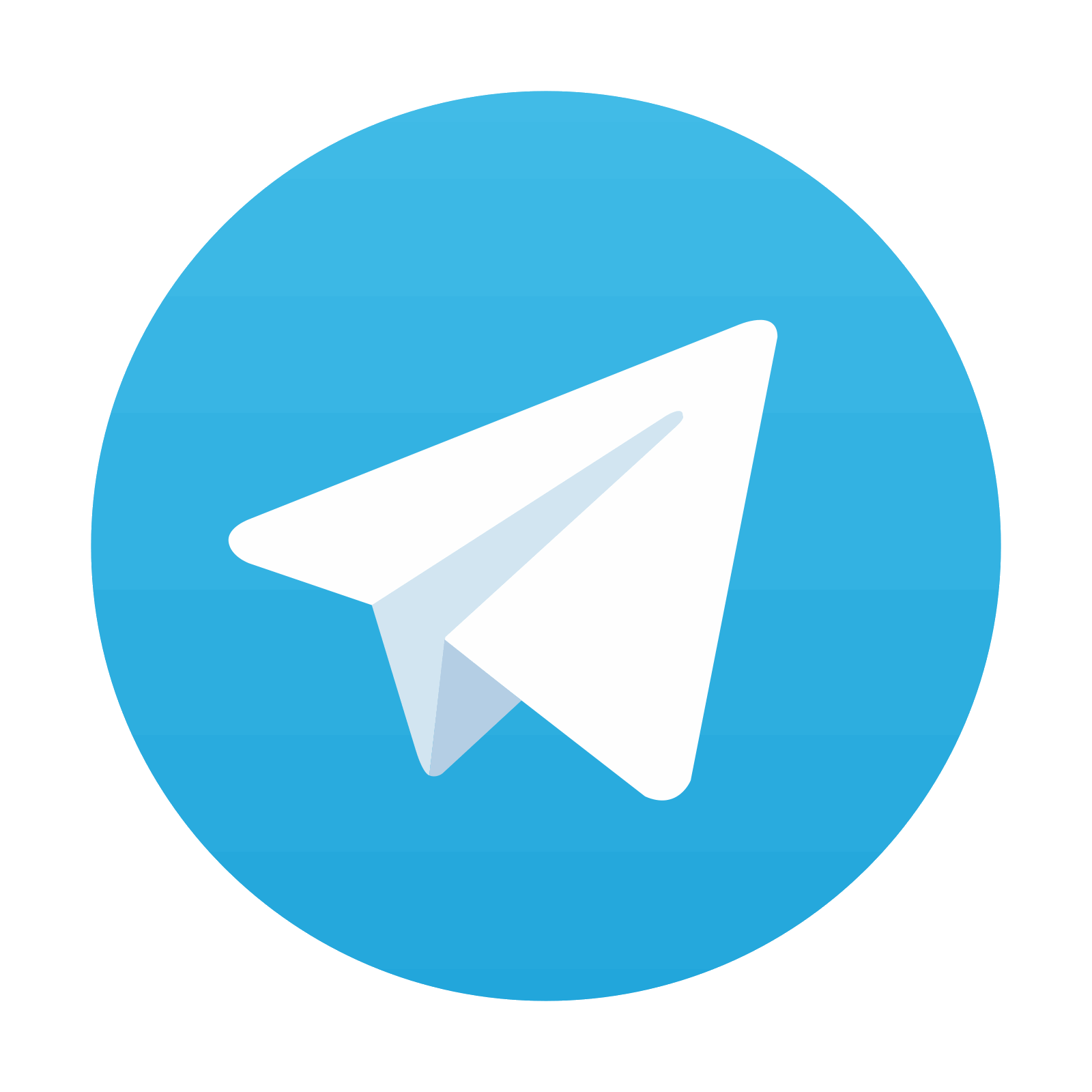
Stay updated, free articles. Join our Telegram channel
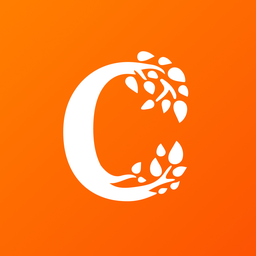
Full access? Get Clinical Tree
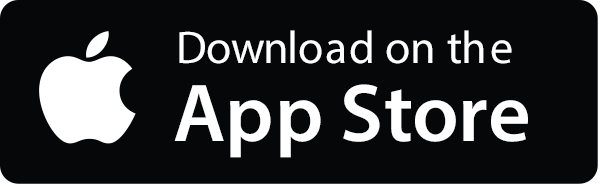
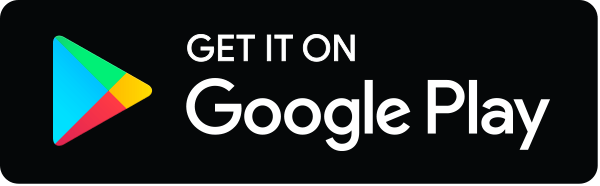