Fig. 1
Example of a delineated volume of interest (VOI). The 3D boundary is approximated by a series of 2D contours (orange). The volume (tumour) is shown in the anatomical context of the patient’s skull, extracted from the corresponding planning CT
The contours can be defined on different types of images and play varying roles in hypoxia imaging for RT, as summarised in Fig. 2.
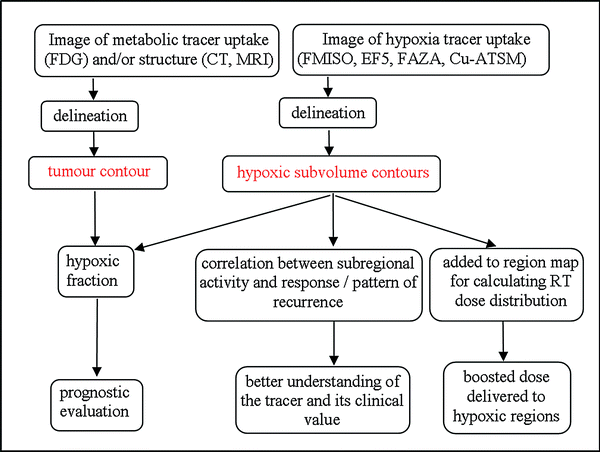
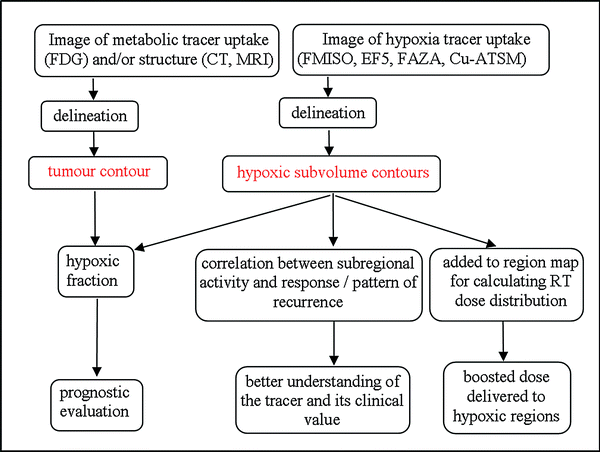
Fig. 2
Flow diagram of the roles of contouring in hypoxia imaging for radiation therapy planning. Contours are used to delineate tumours and hypoxic subvolumes, and enable calculation of predictive measures or radiotherapy dose distributions
A whole tumour may be visible in a structural image such as CT (Rasey et al. 1996; Grosu et al. 2007) and/or functional image such as FDG PET (Komar et al. 2008; Lee et al. 2008). If the whole tumour is delineated, the analyst can calculate a single measure from the voxels inside, such as absolute volume (in cm3) or mean uptake, which help staging and treatment selection. To measure hypoxic fraction (HF), hypoxic voxels are identified in a separate hypoxia image that must be registered to the first image where the contours were defined. HF is then given by the proportion of tumour volume, or number of voxels, containing hypoxic cells. Measure like mean uptake and HF can be used as predictors of treatment outcome, and HF in particular may help in identifying patients suitable for hypoxia-targeting therapy (Rischin et al. 2006).
PET images acquired using hypoxia tracers may reveal hypoxic subvolumes inside a tumour (Grosu et al. 2007; Lee et al. 2008; Rajendran et al. 2006b). Delineating these subvolumes provides spatial information that can be used directly in planning for radiation therapy such as IMRT. The contours are used to boost the dose to hypoxic subvolumes as depicted in Sect. 5.2 in hope to achieve improved tumour control. In practice, tumours and hypoxic subvolumes are delineated manually or by ‘thresholding’ PET images to isolate regions that contrast against the background.
5.1.1 Manual Delineation
In manual delineation, an analyst simply draws round a VOI in a digital image using a computer mouse. For practical reasons, this means creating 2D contours in successive slices as in Fig. 1. Depending on the image analysis software, the user may be able to view the VOI and contours from any viewing angle and refine the delineation to correct for erroneous discontinuities in the tumour surface. The success of manual delineation demands that
the analyst has a great deal of expertise and
the VOI can be seen in the image.
The second requirement makes manual delineation unfeasible for some combinations of cancer type and image modality. For example, in prostate cancer imaging, tumours do not show up in CT images, so PET is used to visualise the VOI. For other disease sites such as lung cancer surrounded by atelectasis, tumours and nodes may be visible on a planning CT and delineated manually on this image, but increased uptake in a complementary PET image (or characteristic pattern in contrast-enhanced CT) still plays a role in confirming that the tumours or metastases are active. For tumour boundaries, the choice of whether to delineate VOIs on a functional image or structural reference image is down to the physician but should be based on knowledge of the limitations of the applied imaging modalities. PET images have lower resolution, so contours would be less precise and there is a risk of overlooking areas with microscopic invasion. However, there is no clear correlation between tissue function and the structures visible in a CT or standard MR image, so contours drawn on these structural images may be misleading e.g. postoperatively when normal tissue planes are lost or in cerebral gliomas which may not delineate well against surrounding brain.
Despite its routine use in structural imaging, manual delineation in biological dose planning is widely viewed as a non-optimal method and may not be feasible at all if hypoxic subvolumes are targeted. This is due to often poor signal-to-noise ratio within the tumour and is likely to be inferior to using FDG for defining BTV when normal tissue background has low metabolic activity.
5.1.2 Thresholding and Other Computational Methods
Thresholding has been preferred to manual delineation in hypoxia-directed planning of RT. This method divides an image into voxels whose intensities are above or below a given value—the threshold. In nuclear imaging, this can automatically define a VOI if the object has a distinct range of intensities. For example, in PET imaging, uptake values above a certain threshold may suggest that the voxel belongs to a BTV. Figure 3 shows the relationship between uptake values and the spatial location of a hypoxic region in an EF5-PET/CT study of head and neck cancer.
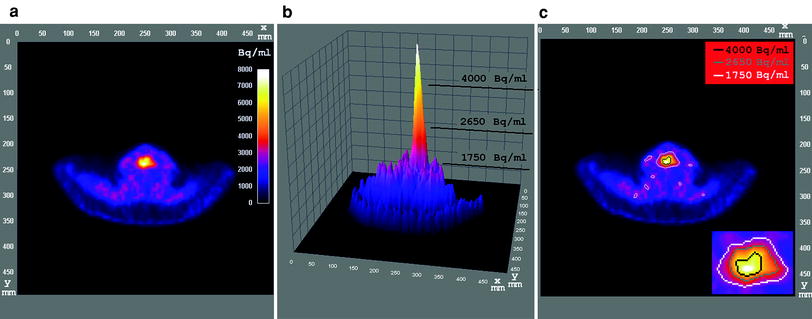
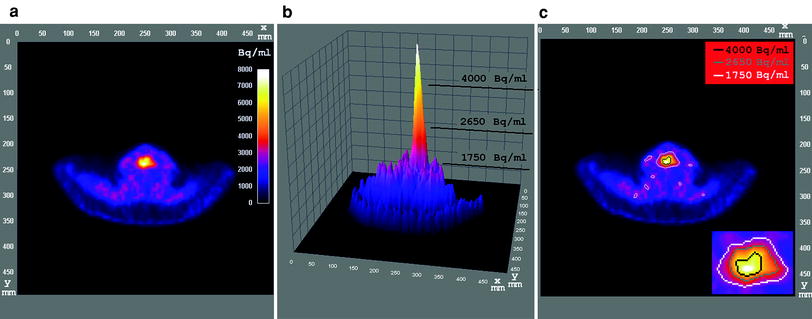
Fig. 3
[18F] EF5 PET image, with 500 mm × 500 mm field-of-view, taken from a head and neck study of a patient with laryngeal cancer (stage IV). a Axial cross section of the lower neck, colour coded according to original PET uptake values, showing a hypoxic region (high uptake) in the neck. b 3D plot of the uptake values in (a), showing the relationship between the position of thresholds in uptake values and in the image plane (x,y). Thresholds are demonstrated at 1,750, 2,560 and 4,000 Bq ml−1. c Axial view as in (a), showing iso-contours corresponding to the thresholds in (b)
The uptake values peak near the centre of the VOI, and fall with distance away from the centre. As a result, voxel intensities equal to the threshold form a closed contour in the image plane, also referred to as an ‘iso-contour’. The contoured volume grows with reducing threshold (Fig. 3c), and the lowest threshold delineates disjoint regions that do not belong to the VOI.
Iso-contours are fast to produce and precisely repeatable. However, the choice of threshold is in practice subjective as there is not one certain value that corresponds to a BTV in all applications or for all patients and images. In particular, the range of uptake values in every PET image is affected by the injected tracer activity as well as many confounding factors of the patient, imaging device and acquisition protocol.
In hypoxia imaging, it is common to work with normalised uptake, where the effect of confounding factors is reduced by dividing values in the whole image by a normalising constant. The constant should quantify the uptake in a reference region, which serves as a biological ‘background’ where the tracer is not trapped. Common choices for the normalising constant are the maximum or mean uptake, calculated over the reference region. The resulting ratio, such as ‘tumour-to-muscle’ or ‘tumour-to-blood’ uptake, is then thresholded. Table 1 shows the reference regions used in different hypoxia studies.
Table 1
Examples of hypoxia studies that use different thresholding schemes for target delineation
Reference | Study area | Hypoxia tracer | Reference region | Threshold(s) |
---|---|---|---|---|
Rasey et al. (1996) | Various | FMISO | Blood | 1.4 |
Chao et al. (2001) | Head and neck | Cu-ATSM | Muscle | 2 |
Eschmann et al. (2005) | Head and neck | FMISO | Muscle | 50 % |
Lung | Mediastinum | |||
Rajendran et al. (2006a) | Gliomas | FMISO | Blood | 1.5 |
Souvatzoglou et al. (2007) | Head and neck | FFAZA | Muscle | 50 % |
Nehmeh et al. (2008) | Head and neck | FMISO | Blood | 1.2 |
Lin et al. (2008) | Head and neck | FMISO | Blood | 1.3 |
Komar et al. (2008) | Head and neck | EF5 | Muscle | 1.5 |
Lee et al. (2008) | Head and neck | FMISO | Blood | 1.3 |
Grosu et al. (2007) | Head and neck | FFAZA | Muscle | 1.5 |
It is clear from Table 1 that there is no consensus on the thresholding scheme to use. In some cases, the threshold is given by a percentage of the maximum rather than an absolute value. This may help to further standardise the threshold across different images, but the maximum value is inherently sensitive to image noise. In cases where an absolute threshold is used, values range from 1.2 to 1.5 in tumour-to-blood concentration ratios and from 1.5 to 2 in tumour-to-muscle. The selection of threshold depends on the applied hypoxia marker and reflects the overall signal-to-noise ratio (SNR) and the imaging protocol. Compared to FDG studies, hypoxia markers generally suffer from much lower SNR.
The lack of consensus on the choice of threshold is cause for concern. Small differences can have a large effect on the measures sought by image analysis, such as absolute volume or hypoxic fraction. For example, increasing the threshold on tumour-to-muscle uptake ratio from 1.4 to 1.6 led to a reduction in percentage hypoxic area (an analogue of HF) from 48.9 % down to 16.0 % in Komar et al. (2008). The choice of threshold also affects the shape and volume of the region it defines, as seen in Fig. 3c.
Other computational methods that aim to overcome the subjectivity of thresholding, are seen for the more common imaging of metabolic PET, but are yet to be adapted and validated for hypoxia imaging due to the relative lack of test data in this new field. However, it is expected that these methods will enter hypoxia treatment planning in the future as they promise to reduce not only the inter-observer variability of contouring but the currently excessive amounts of time spent on manual delineation or the selection and editing of iso-contours. In general, methods proposed for automatic tumour contouring outside hypoxia imaging perform computations on higher level information in PET or hybrid images. Examples are the use of image gradients (Geets et al. 2007) and local histograms (Hatt et al. 2009) in PET, the combination of PET and CT information (El-Naqa et al. 2007) or the analysis of time series from dynamic PET (Shepherd and Owenius 2012).
5.2 Dosimetric Considerations
Since high levels of tumour hypoxia have been found to correlate with poor radiation response, the most intriguing approach to increase the therapeutic ratio is dose escalation to the hypoxic volumes in the tumour. Theoretical studies indicate that hypoxia is the dominant factor in determining the optimal dose distribution and that the effect of TCP also depends on the degree of reoxygenation during treatment (Søvik et al. 2007b; South et al. 2009). The dose to the hypoxic subvolume of the tumour has to be escalated by a factor of the OER to achieve the same therapeutic ratio as in oxic cells. The relationship between different oxygenation levels and OER has been mainly defined from in vitro cell survival data and estimated values of the OER have been in the range 2.5–3.5 (Horsman et al. 2009). However, the actual in vivo OER is less than or equal to 1.5 derived from data of cervix carcinoma (Carlson et al. 2006) and the range of proposed dose escalation factors in biological dose escalation strategies has varied between 1.2 and 1.5 (Lin et al. 2008; Søvik et al. 2009). Hence, more clinical data is required to define the OER in vivo and more information of the quantification of the OER from biological images before the start of the treatment is needed.
5.2.1 Dose Escalation Strategies
Different dose escalation strategies have been used in hypoxia-targeting studies. One strategy is to selectively boost the dose to the hypoxic volumes while keeping the integral target dose constant (Søvik et al. 2007a, b; Petit et al. 2009). The other approaches are to give an additional boost dose to the hypoxic volume (Popple et al. 2002; Tomé and Fowler 2000; Lin et al. 2008) or to plan the dose escalation with respect to target coverage at constant normal tissue toxicity (Thorwarth et al. 2007). An example of the latter can be seen in Fig. 4, where the dose in the hypoxic subvolumes defined on EF5 PET/CT images is escalated without increasing the dose to critical structures when compared to the conventional treatment plan. The difference in dose in the delineated hypoxic subvolumes and the dose equivalence in critical structures can be seen from the dose volume histograms in Fig. 5. The dose escalation to the resistant cells can be realised by DPN or by discretising the hypoxia parameters into a few discrete levels. In DPN, the dose prescription in each voxel is based on the voxel intensity of the hypoxia image, whereas with level discretisation, subvolumes are divided into a number of levels with the same dose. The number of levels used in treatment planning is certainly dependent on the hypoxic distribution within the target, but increasing the number of levels beyond four yields only little additional benefit (South et al. 2009; Søvik et al. 2007a).
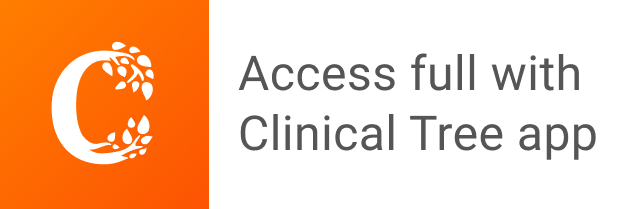