Fig. 1
Principle of BLI
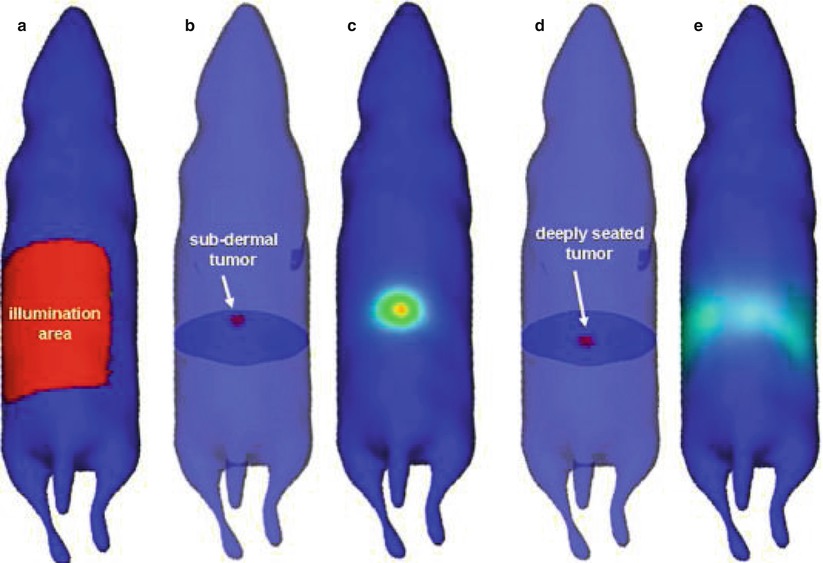
Fig. 2
Non-linear relation between tumour depth and optical signal (Figure from Leblond et al. [114])
Fluorescence Imaging
Fluorescence (i.e. optical) imaging (FLI) can be either direct or indirect. Direct FLI is performed by integrating a transporter gene, often that of green fluorescent protein (GFP), into the organism. The fluorescent protein that is produced upon transcription of the gene can subsequently be imaged.
Indirect FLI does not require genetic alterations, as imaging is performed using an externally administered fluorescent substance, such as a fluorescently labelled antibody or small molecule or simply by injecting solely the fluorescent compound. Upon binding to its target receptor, the fluorescently labelled agent will yield a signal that is specific for cells that carry the target. Nontargeted fluorescent dye such as indocyanine green (ICG) can be used in perfusion studies and to detect the sentinel lymph node in cancer surgery [1–4]. Upon excitation of the fluorescent dye with light of the appropriate wavelength, the electrons shortly reach a higher energy state by absorbance of one or more photons. This phase ends with the emission of a photon with lower energy and thus a slightly higher wavelength and return of the electron into its stable state (Fig. 3). The emitted light can be captured with a fluorescence camera system.
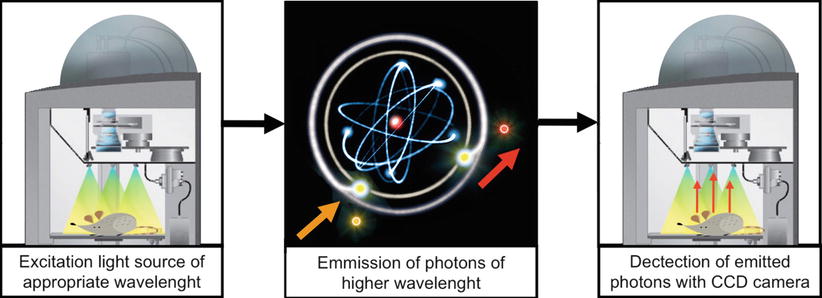
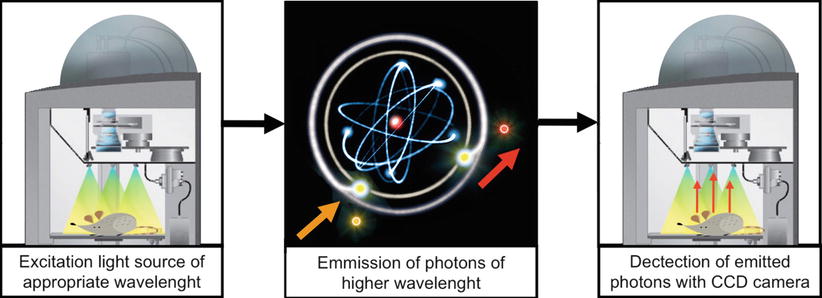
Fig. 3
Principle of FLI
As mentioned above, light in the NIR range is most suitable for in vivo imaging. There are multiple examples of in vitro FLI using an agent that emits in the visible spectrum, mostly based on fluorescein, but results in vivo are far more sensitive when using a NIR fluorescent agent. This light is not visible without sensitive charge-coupled device (CCD) cameras, most of which now combine colour imaging and FLI. Similarly to BLI, both images can be superimposed to help accurately locate the fluorescent signal in the organism (Fig. 4). FLI shares the disadvantages of BLI of having limited tissue penetration and the inability to draw conclusions about tumour size and depth.
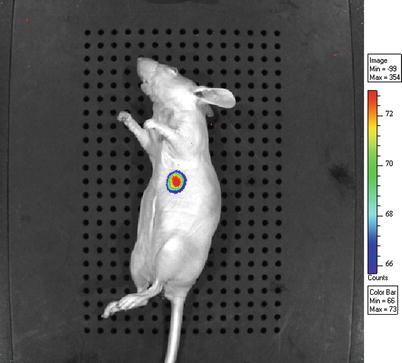
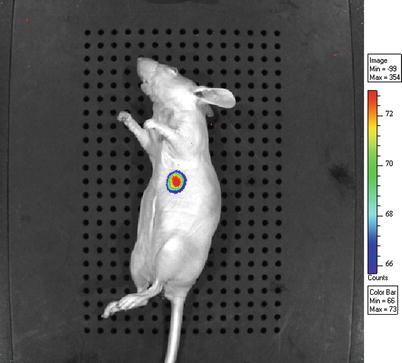
Fig. 4
BLI signal superimposed on color image (depicted in greyscale)
Fluorescence Epi-illumination and Transillumination
Most imaging studies are performed using epi-illumination, meaning that the light source for excitation is on the same side of the subject as the CCD camera that translates the emitted signal to an image. Conversely, transillumination refers to a light source opposite from the camera, thus passing the light through the image (Fig. 5) [5]. A shortcoming of the latter is the limited penetration of photons through tissue; adipose tissue in particular, thus making it unsuitable for clinical application. Epi-illumination is often used in preclinical studies because of its simplicity and because scattering and penetration are of limited concern in small animals. In clinical studies in which the subject is located either subcutaneously or in direct view of the CCD camera, for instance, during surgery, epi-illumination is the technique of choice.
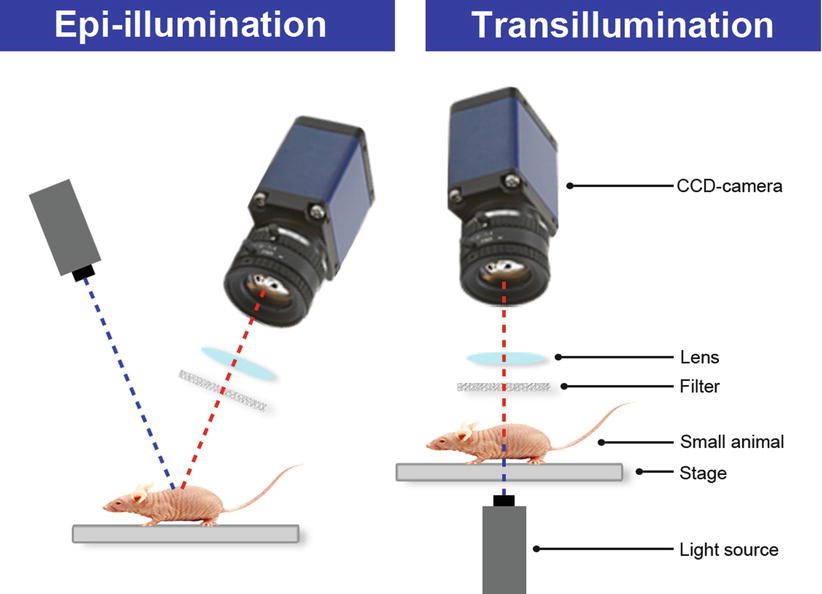
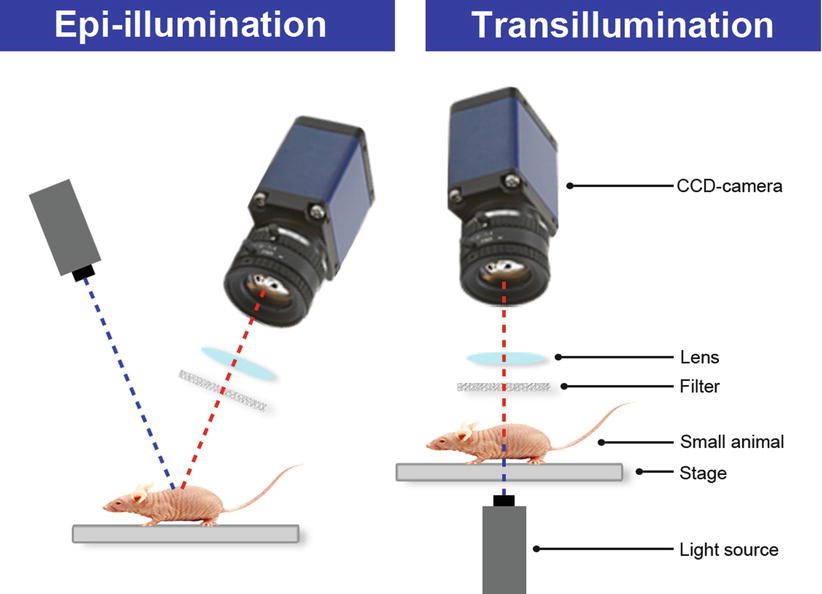
Fig. 5
Epi- and transillumination
Fluorescence Molecular Tomography
In fluorescence molecular tomography (FMT), the emitted light is measured in different directions. A mathematical calculation transfers this data into a 3D image of the fluorescent or bioluminescent signal within the small animal. Advantages over planar imaging are the ability to calculate volume and depth of the light-emitting tissue of interest. FMT is not used in clinical imaging as light propagation through tissue is limited.
Photoacoustic Imaging
A relatively new technique is photoacoustic imaging, in which the fluorescent agent is excitated by light similar to FLI, whereupon thermal expansion causes the emission of ultrasound waves (Fig. 6) [6]. Similar to light propagation, ultrasound waves also suffer from scattering, albeit to a lesser extent. Due to this greater stability of the signal, photoacoustic imaging allows for deeper imaging than FLI, with a current penetration depth of more than 1 cm [7].
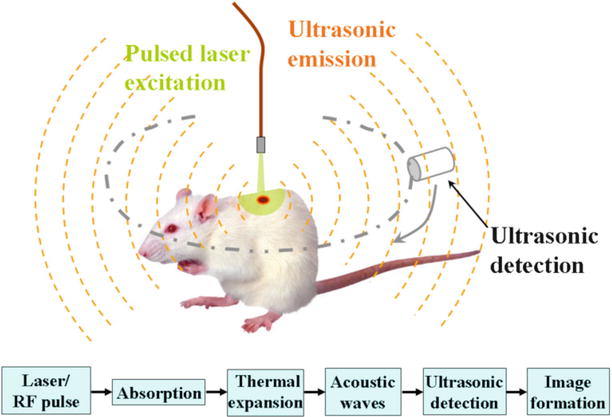
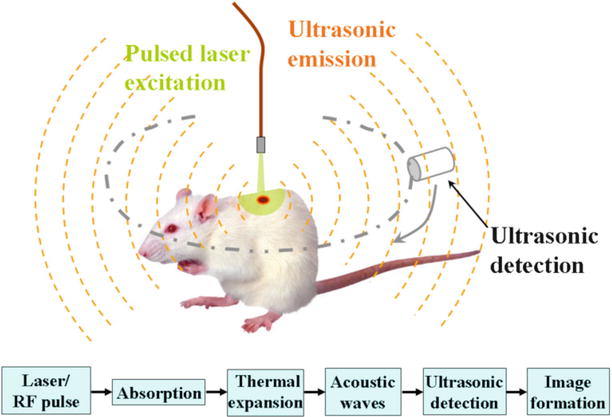
Fig. 6
Principle of photoacoustic imaging (Wikipedia)
Although photoacoustic imaging is an attractive technique for small animal imaging and for imaging of relatively superficial tumours, its value for diagnosis of ovarian cancer is probably low, as tumours and affected lymph nodes are located deep inside the pelvis.
Photodynamic Therapy
The last application of molecular imaging to be mentioned is photodynamic therapy (PDT), in which light is used to induce cell death. This is strictly not an imaging modality but is closely related as light is being used for a tumour-targeted therapeutic approach. A photosensitizer is activated by light of a specific wavelength, whereupon it initiates, in the presence of oxygen, a chemical reaction in which singlet oxygen (1O2) and oxygen radicals are generated. These in turn provoke immediate cell damage in the form of apoptosis or necrosis or indirect cell death through vascular damage, ischemia and inflammatory reactions [8]. A natural photosensitizer is protoporphyrin IX (PPIX), produced by cells during the haem cycle. Synthetic photosensitizers and prodrugs are increasingly being developed, with favourable characteristics for clinical application. The major disadvantage of PDT is phototoxicity in other tissues than the tumour, leading to strict living rules for patients and requiring a very well-designed illumination area. In case of a sprouting tumour, or a tumour close to vulnerable structures or organs, illumination can easily cause collateral damage, resulting in undesirable side effects.
Photo-immunotherapy (PIT) is a derivative from PDT in which the problem of harming surrounding structures is largely overcome. The photosensitizer is conjugated to a tumour-specific antibody, thus leading to tumour cell death only while leaving healthy tissue unharmed [9]. Unbound photosensitizer does not cause any phototoxicity.
Radioguided Imaging
Imaging plays an important role in cancer diagnosis and treatment follow-up. PET, SPECT, MRI, CT and combinations of these techniques are indispensable in clinical practice, and extensive research is performed to continuously improve sensitivity and specificity rates.
Positron Emission Tomography
In positron emission tomography (PET) scanning, gamma rays are used to detect a radionuclide that is taken up by certain molecules in the body. The most often used tracer is fludeoxyglucose conjugated to fluorine-18 (18F-FDG), which is a derivative of glucose that enables visualization of metabolically active cells and cell processes. Tumours and metastases require large amounts of glucose to support their high expansion rate; however, glucose uptake is not cancer specific. Tumour-specific PET scanning, using an antibody bound to a radioactive label, is now increasingly being performed in order to discriminate healthy tissue from malignant processes [10].
Single-Photon Computed Tomography
Similar to PET, single-photon emission computed tomography (SPECT) makes use of gamma rays to visualize the distribution of a radioisotope within the body. Its resolution of 1 cm performs worse than PET; however, the technique is significantly less expensive. Targeted SPECT can be performed similar to targeted PET, by using radiolabelled antibodies. Both PET and SPECT are often coupled to a CT scan for assessment of exact anatomical localization of lesions.
Magnetic Resonance Imaging
Magnetic resonance imaging (MRI) produces a large magnetic field that forces protons (H+; abundantly present in water molecules) in the body to align in the same direction. Short exposure with an electromagnetic field makes the protons flip and then realign, in which electromagnetic radiation is produced that is measured and processed into an image. MRI contrast agents, most based on gadolinium, are used to improve the signal from tumours, blood vessels or other specific tissues or structures. For several years, the use of iron oxide particles was considered feasible for imaging of solid tumours; however, most tracers are no longer produced because of safety matters.
Conjugation of ligands to gadolinium with the use of a chelator, often DTPA, yields tumour-specific MRI contrast agents that are likely to improve diagnostic accuracy [11].
Requirements for Molecular Imaging
In molecular imaging, the use of a sensitive detection system is essential to obtain adequate signal-to-noise ratios. Moreover, except in the case of bioluminescence and intrinsic fluorescence, a fluorescent agent with or without a target is required as well as an excitation light source of the appropriate wavelength.
Fluorescent Agent
The fluorescent agent can be either naturally expressed by a cell or organism or exogenously administered. Indirect fluorescence can range from staining of cells in vitro with, for instance, DAPI, in order to detect nuclei by fluorescence microscopy, to intraoperative detection of metastases in patients.
Virtually all objects emit fluorescent light to a certain level, albeit not visible by the naked eye because the signal is by far not strong enough to compete with the visible light. Fluorescence in the visible light spectrum (400–750 nm) is less suitable for imaging purposes, as background fluorescence is high. In contrary, imaging in the near-infrared (NIR) spectrum (750–1000 nm) yields a high signal-to-background ratio, as autofluorescence is minimized (Fig. 7). In living organisms, light <700 nm is to a great extent absorbed by haemoglobin, while wavelengths longer than 950 nm suffer from absorption by water (Fig. 8). Fluorescent agents that emit light in the NIR range are therefore most suitable for in vivo application [5]. Lower wavelengths cause damage in DNA and are therefore not used.
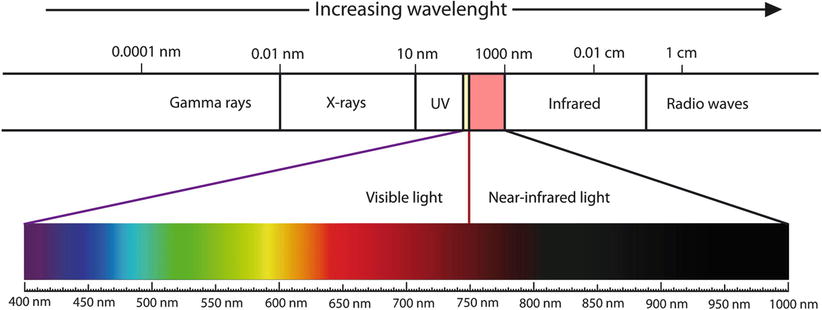
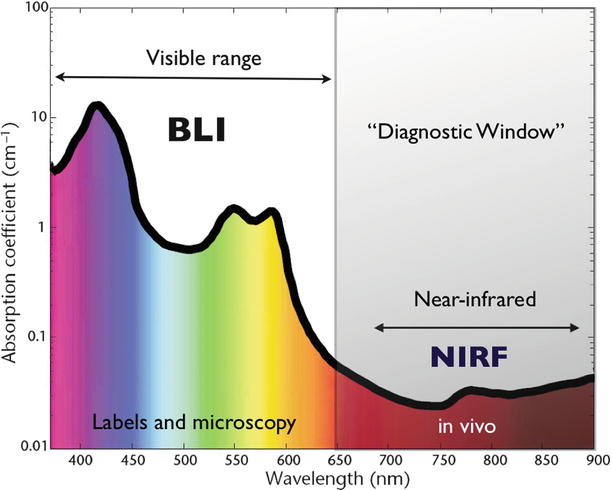
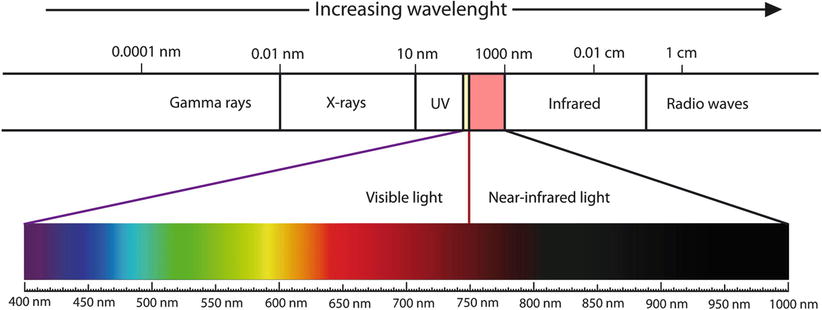
Fig. 7
NIR spectral range
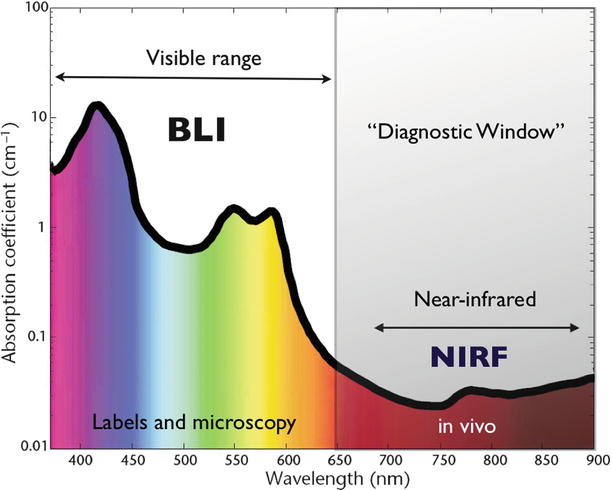
Fig. 8
Absorption in NIR spectral range
There are three types of fluorescent agents. First, non-specific fluorescent dyes that do not bind to cells but flow along with bodily fluids (blood, lymph or gall). These can be used for perfusion studies or to identify the sentinel lymph node in cancer. Second, targeted agents are fluorescent dyes that are conjugated to a small molecule or antibody that is directed at a certain biomarker. This allows for tissue-specific or tumour-specific imaging. Third, activatable probes do not emit light until cleaved by the appropriate enzyme (Fig. 9). Currently, only three fluorophores are approved for clinical use: indocyanine green (ICG), fluorescein isothiocyanate (FITC) and IRDye800CW [12].
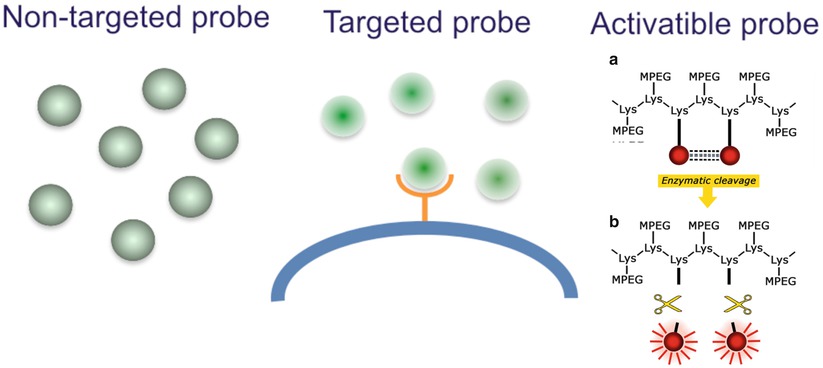
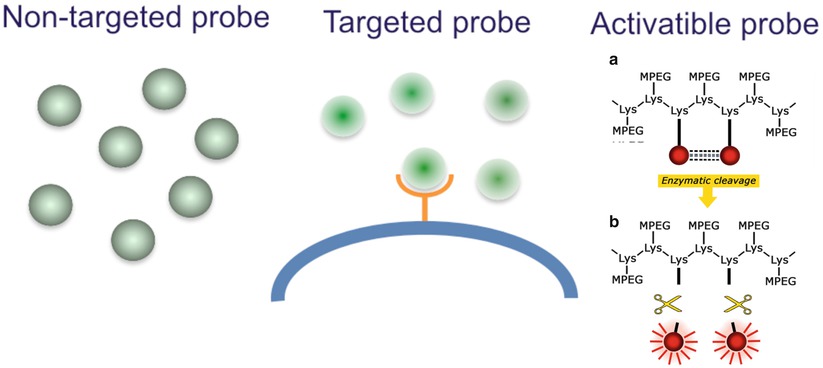
Fig. 9
Fluorescent agents: non-specific, targeted, activatable
Light Source and Imaging System
Because the human eye is incapable of detecting NIR light, a specialized imaging system is needed to transfer the fluorescence data into an image. Generally, the imaging system consists of one or multiple laser beams of a specific wavelength for excitation. The subsequently emitted signal is captured by a series of lenses and filters and processed by a sensitive charge-coupled device (CCD) camera. With the help of mathematical algorithms and software, most systems provide both a colour image and a fluorescence image. Some, but not all, are capable of superimposing the two, yielding an accurate representation of the fluorescent signal in the background. The list of imaging systems is long, with both preclinical and clinical cameras on the market (Fig. 10). In the following sections on preclinical and clinical imaging, the relevant systems will be mentioned.
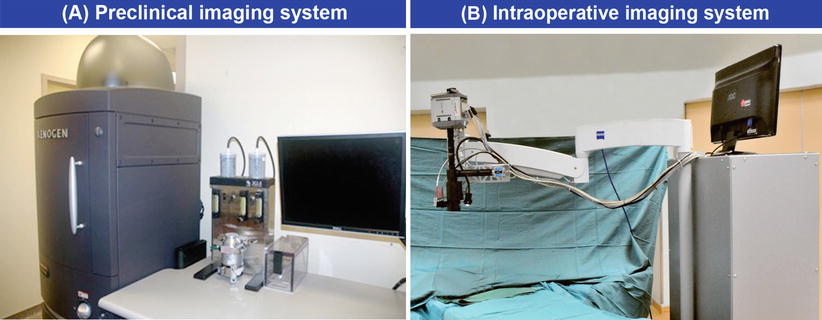
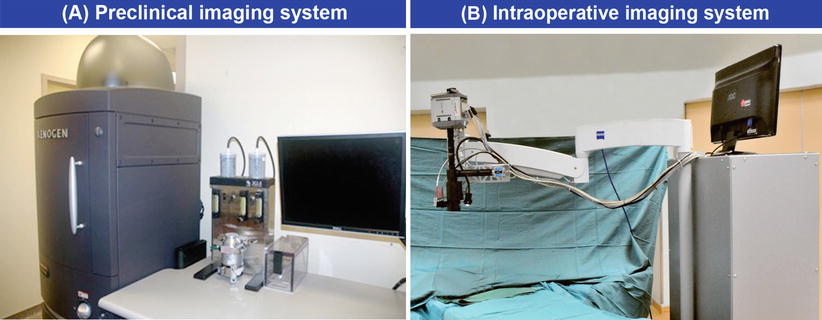
Fig. 10
Preclinical (a) and clinical (b) imaging systems
Targeted Imaging in Ovarian Cancer
Target Selection
In cancer treatment, the goal is to differentiate tumour cells from healthy cells. This requires the identification of tumour-specific biomarkers that can serve as targets for either fluorescent agents or targeted (chemo)therapy.
No validated system to select biomarkers has been designed yet, but a number of favourable characteristics can be mentioned [13]. (I) Tumour selectivity can only be achieved if the biomarker is present on malignant cells and absent or only weakly expressed by healthy cells, thus yielding a high tumour-to-normal ratio. (II) Both targeted imaging and (chemo)therapy require the majority of tumour cells to express the biomarker in a diffuse pattern (III). Although cell death can induce apoptosis in neighbouring cells, heterogeneous expression can lead to parts of the tumour being missed because overall expression is not sufficient. From an economical perspective, biomarkers that are present on the majority of tumours (i.e. in a majority of patients) are preferable. (IV) An extracellular or transmembraneous localization facilitates binding, while internalization (V) leads to accumulation and thus a higher fluorescent signal or a stronger chemotherapeutic effect. (VI) Enzymatic activity is needed when using activatable probes. (VII) For imaging purposes, a biomarker that has already been used in vivo and has shown no toxicity is favourable, as this may shorten the road to clinical application.
Targets in Ovarian Cancer
In ovarian cancer, numerous targets have been described for molecular imaging. However, most are far from implementation in the clinical setting due to lack of toxicity data. A small number of targets have been validated in either preclinical or clinical studies. In this section, only the biomarkers that are suitable for imaging are discussed.
CA-125
Tumour marker CA-125, or MUC16, is expressed in more than 95 % of non-mucinous stage III–IV epithelial ovarian tumours and is used for diagnosis as well as for treatment evaluation and follow-up [14]. Oregovomab is a monoclonal antibody directed at both membrane-bound and soluble CA-125 and has been studied in a number of preclinical and clinical pilot studies. Although the toxicity profile shows that the antibody is safe in the short term and after a 5-year follow-up, no clinical survival benefits can be shown, leaving the exact value of oregovomab unclear [15]. However, an antibody approved for clinical use with a target that is overexpressed in 95 % of tumours may have potential value as a fluorescent agent when conjugated to a near-infrared dye. In the case of oregovomab, this is still entirely theoretic but plausible.
Carbonic Anhydrase IX
Carbonic anhydrase IX (CA-IX) is a hypoxia-related transmembrane protein that is present in several solid tumours and upregulated in ovarian cancer [16, 17]. Staining studies show mainly upregulation in hypoxic areas. Although CA-IX inhibitors have been used as PET imaging agents [18], the focal expression may hamper intraoperative optical imaging as non-hypoxic areas may be overlooked. Nonetheless, CA-IX could turn out to be a feasible target in other diseases, such as vulvar cancer, in which lymph node metastases were found to overexpress the marker [19]. Molecular targeting of CA-IX has already been performed in a mouse model [20]. A number of monoclonal antibodies are now available for preclinical testing; possibly one of these will find its way to the clinic for both therapeutic and imaging purposes.
CXC Chemokine Receptor 4
Chemokine receptor pairs play an important role in tumour metastasis, which is thought to start with the loss of adhesion molecules and degradation of the extracellular matrix. The CXC chemokine receptor 4 (CXCR4) and its chemokine CXCL12 or stromal cell-derived factor 1 (SDF-1) are known to be involved in dissemination of several solid tumours [21–23]. Although not specific for ovarian cancer, MMP and CXCR4-CXCL12 are indicative of metastatic tissue and are therefore worth exploring as targets for imaging, as complete cytoreduction includes removal of metastatic tumour nodules.
The CXCR4-CXCL12 pair seems to be more important than other chemokine receptor pairs in ovarian cancer and is associated with a worse prognosis [24]. This pathway promotes chemotaxis of tumour cells to the metastatic site, where it subsequently facilitates hatching and proliferation. Upon binding to CXCR4, CXCL12 is internalized, after which the receptor is recycled and transported back to the cell membrane within 15 min. Staining studies in ovarian cancer showed CXCR4 staining in 59 % of tumour cells and CXCL12 staining in 91 % of tumour cells, whereas no expression was found in healthy cells [25]. CXCL12 is also found in ascites, where it contributes to peritoneal dissemination.
Imaging of the CXC receptor has been performed by using a CXCR4-directed fluorophore in in vitro models [26, 27]. In vivo imaging in a brain tumour mouse model showed tumour uptake of a CXCR4-radiolabelled monoclonal antibody [28]. Furthermore, the CXCR4 antagonist ADM3100 conjugated to either 99mTc or 64Cu proved useful in PET localization of tumour xenografts in mice [29, 30]. Due to favourable dosimetry results, introduction into the clinic may be close. As described above, optical imaging requires conjugation of a biomarker to a fluorescent agent and is more likely to be successful once the marker has already been used for imaging. The CXCR4-CXCL12 axis may therefore be an interesting target for fluorescence imaging in the (near) future.
Epidermal Growth Factor Receptor
The epidermal growth factor receptor (EGFR; HER1) plays a significant role in ovarian cancer, as most epithelial tumours express EGFR to some extent, leading to low survival rates [31–34]. Upregulation of EGFR leads to cell proliferation, differentiation, migration, adhesion, protection from apoptosis and malignant transformation [35]. HER1 and HER2 are expressed in 35–70 % and 11 % of epithelial ovarian tumours, respectively. HER1 is not only expressed by cancer cells but also by normal epithelial cells. However, expression rates in tumour are a reported 15–20 times higher, yielding a tumour-to-normal ratio that is high enough for tumour-selective imaging.
The anti-HER1 monoclonal antibody cetuximab is approved for chemotherapeutic treatment of metastatic colorectal cancer, while the anti-HER2 trastuzumab is being used for breast cancer treatment and for metastatic gastric cancer and panitumumab is approved for metastatic colorectal cancer. Dual-labelled radioactive/fluorescent trastuzumab showed comparable uptake in an ovarian cancer mouse model, indicating a possible role for clinical application [10]. Panitumumab-800CW was used for imaging of primary tumours and lymph node metastases smaller than 1 mm in a head-and-neck squamous cell cancer model, showing improved detection compared to panitumumab conjugated to non-specific IgG [36]. Furthermore, panitumumab-800CW and trastuzumab-ICG have been evaluated in an orthotopic breast cancer model [37]. Cetuximab and panitumumab imaging has been described in animal models, but none of these antibodies have been tested in a clinical imaging setting yet [38, 39]. The fact that there are at least three FDA-approved antibodies targeting EGFR, some with promising preclinical imaging data, may lead to faster translation of fluorescent agents into the clinic, for optical imaging of possibly both breast and ovarian tumours.
Epithelial Cell Adhesion Molecule
The epithelial cell adhesion molecule (EpCAM) is a cell surface receptor involved in cell adhesion and is expressed on most epithelial cells. Several solid tumours overexpress EpCAM. Primary epithelial ovarian tumours, metastases and recurrent tumours all show a significantly higher expression than normal ovarian tissue, in which expression on metastatic tumour cells is significantly higher than on malignant cells of the primary tumour [40]. High EpCAM expression is associated with increased tumour cell migration and portends poor survival [41].
Preclinical imaging has been described using an antibody fragment targeting EpCAM conjugated to a radionuclide for PET imaging, as well as near-infrared EpCAM-directed probes in breast and prostate cancer [42–44]. Even more interestingly, two FDA-approved monoclonal antibodies targeting EpCAM are now available; edrecolomab and catumaxomab. Although edrecolomab showed no clinical benefit in colorectal cancer, it has proven safe in clinical application. Both of these monoclonal antibodies bear potential for intraoperative imaging application, as the overexpression of EpCAM yields a high tumour-to-normal ratio. The value in vivo needs yet to be studied.
Folate Receptor
The folate receptor has been identified as a promising target in epithelial ovarian cancer (EOC), as 72–97 % of the tumours overexpress the alpha-isoform of the receptor (Fig. 11) [45, 46]. Furthermore, it is known that expression of FR-α is not influenced by adjuvant chemotherapy, allowing for FR-targeted imaging during primary as well as interval debulking surgery [47]. Its substrate folate, the natural form of folic acid in the body, is a small molecule that is suitable to deliver either imaging agents or chemotherapeutic drugs into the cell. Folate has a very high affinity for FR-α rather than for physiologic folate carriers on healthy cells, and the folate conjugate is easily internalized into the cell where it stays stable for hours in an endosome, before being broken down. Further advantages are that folate is inexpensive, non-toxic and easily to conjugate.
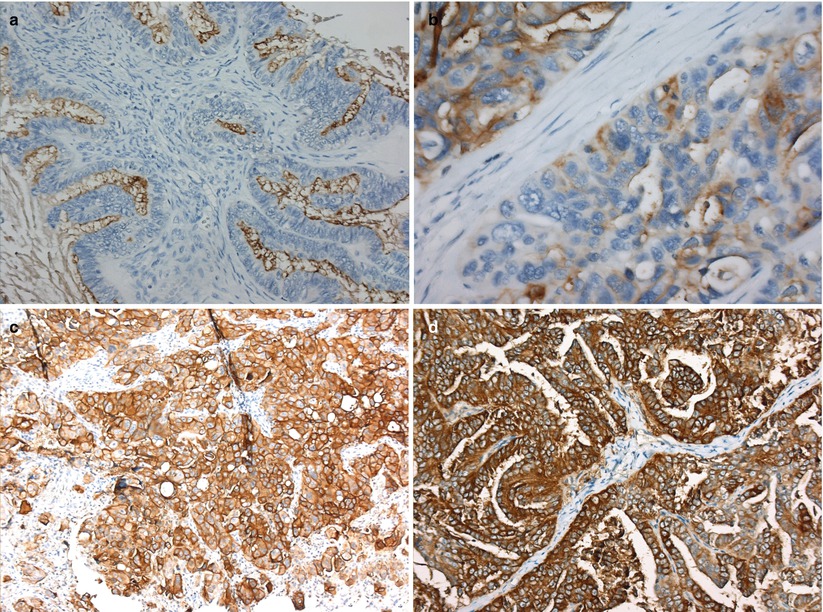
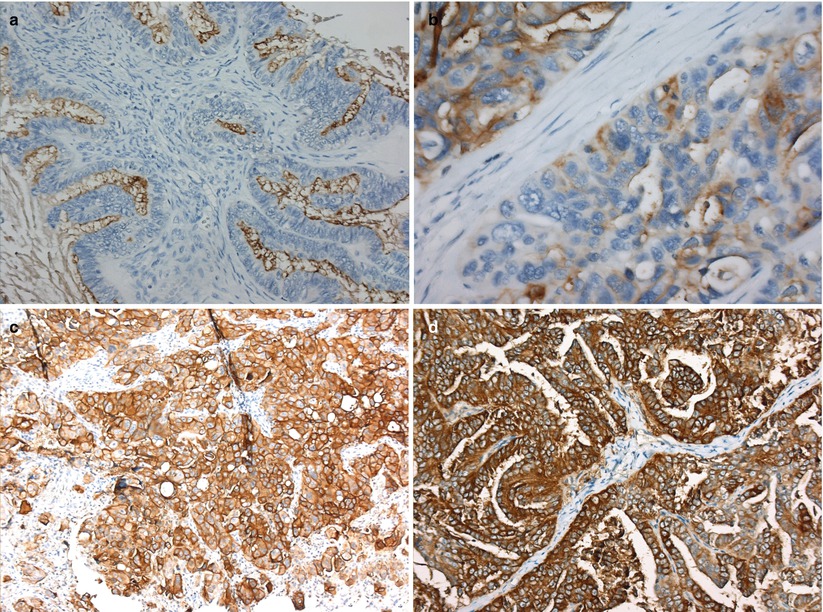
Fig. 11
Folate receptor-alpha expression in serous ovarian cancer. (a) No expression (negative), (b) weak expression, (c) moderate expression, (d) strong expression (Crane et al. [47])
A number of radioactive and chemotherapeutic agents targeting the FR-α have been developed [48–50]. Nuclear imaging with 111In-DTPA-folate shows uptake of the tracer in ovarian cancer and in the kidneys, where FR-α is physiologically expressed [51, 52]. A second nuclear tracer, 99mTc-folate, has also shown clear uptake in FR-α-positive tumour tissue [53, 54]. The FR-α-targeted monoclonal antibody farletuzumab is now in phase III trials and seems to be safe and effective in the treatment of platinum-resistant tumours [55, 56]. As FR-α is not only overexpressed by ovarian cancer cells but also by other malignancies, FR-targeted optical imaging is increasingly being studied. Several groups have reported the use of nanobodies to either visualize tumour cells or transport drugs into the tumour; however, this is still limited to preclinical research [57].
The only clinical study using FR-targeted optical imaging was presented in 2011 [58]. In this study, folate conjugated to fluorescein isothiocyanate (FITC) was used as a fluorescent agent. Patients with suspected ovarian cancer received an injection of folate-FITC 2 h prior to surgery, allowing for the agent to be taken up by the tumour. During the procedure, a custom-built intraoperative camera system was used to visualize FR-positive tumour cells. Peritoneal metastases as small as 1 mm were found with the aid of fluorescence (Fig. 12). All fluorescent tissue was proven to contain tumour cells, whereas nonfluorescent tissue did not contain malignant cells, and healthy tissue did not emit any fluorescence. This study shows the potential of intraoperative fluorescence imaging, as a significantly higher number of tumour spots were found based on fluorescence compared to surgery without optical imaging. The dye used, FITC, has an emission wavelength that lies just within the visible spectrum, and imaging is to a certain amount hampered by background fluorescence. FR-targeted agents using a NIR dye is expected to yield even better results.
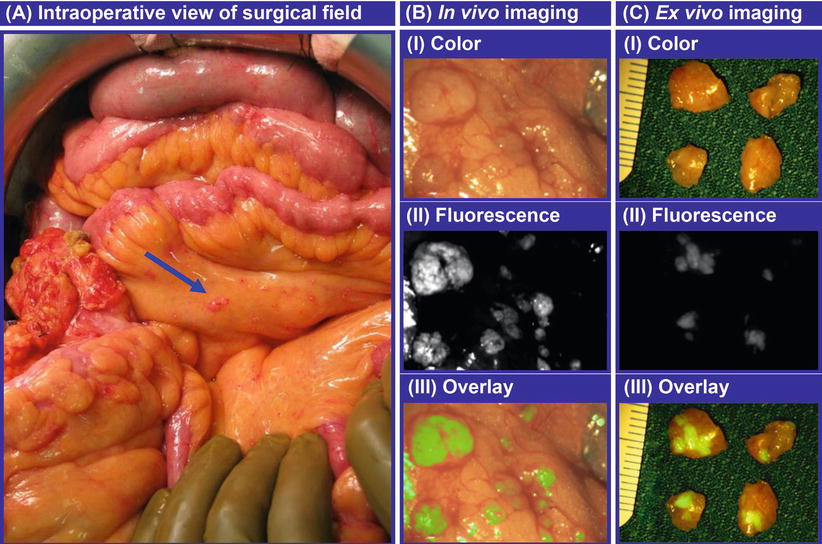
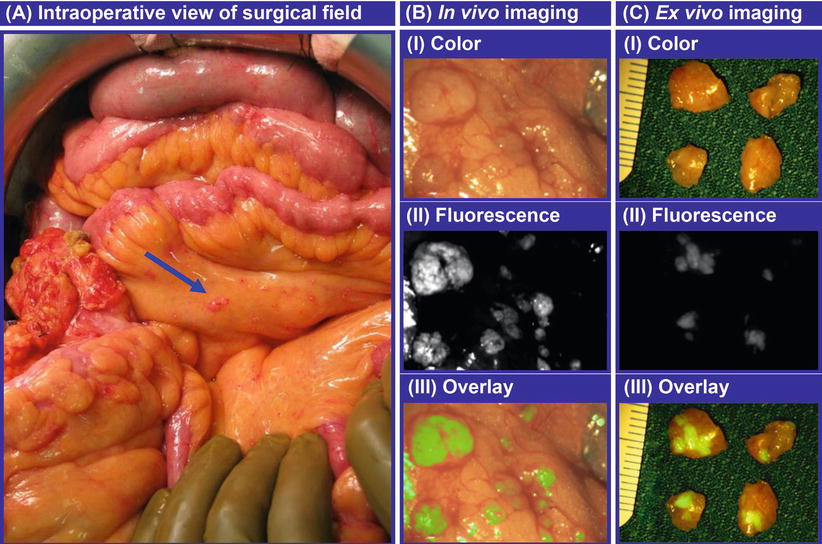
Fig. 12
Intraoperative fluorescence imaging in ovarian cancer. (a) The arrow points towards a tumor deposit of 1 mm that was detected with fluorescence imaging, (b) a close-up image of a tumor nodule, showing the color image (I), the fluorescence image (II) and the fluorescence image superimposed on the color image (III), (c) a close-up image of four excised tumor nodules, showing the clear distinction between healthy tissue (no fluorescence) and tumor deposits (fluorescent) with a resolution of <1 mm
Integrins
The integrin family comprises a great number of cell adhesion molecules including transmembrane glycoproteins that bind to a large variety of ligands, usually containing the RGD tripeptide (Arg-Gly-Asp). Multiple pathways can be activated upon binding: regulating cell proliferation, differentiation, apoptosis and migration. Almost all cells express integrins but generally not more than a few subtypes. In the normal ovary, integrins are thought to play an important role in follicular development and cell differentiation [59]. In tumorigenesis, integrins and matrix metalloproteinases (see next) activate and regulate each other, thus creating a positive feedback loop towards extracellular matrix degradation and metastasis. In the last decade, the importance of integrin subtype and vitronectin receptor αvβ3 has become clear, as it binds a specific metalloproteinase containing an RGD-sequence and as such creates a clear pathway for dissemination. Subsequently, an alternative pathway of cell migration is activated in which cell adhesion is disrupted and tumour cell invasion is facilitated. Furthermore, αvβ3 promotes angiogenesis, essential for tumour growth.
Integrins, and especially αvβ3, are increasingly being used for imaging purposes. Several studies report of αvβ3-specific binding in vitro and in vivo, some using a dual-labelled optical and PET imaging probe, some using only a near-infrared optical agent [60–64]. A few attempts have been made to target αvβ3 in humans, mainly with PET and SPECT tracers, in which tumours were clearly detected in contrast to liver and bone lesions [64–66]. The monoclonal antibody etaracizumab was shown in clinical phase 0/I studies to have an acceptable toxicity profile and might have potential as an imaging agent [67, 68].
Matrix Metalloproteinase
Matrix metalloproteinases (MMPs) facilitate tumour dissemination by degrading the extracellular matrix. Several MMP subtypes are overexpressed in ovarian cancer, among which MMP1, MMP2, MMP7 and MMP9 [69–71]. CXCL12 activates MMP2 and MMP9 in ovarian tumours, thus directly and indirectly promoting metastasis.
Near-infrared fluorescence imaging of MMP activity can be performed using ‘smart activatable probes’ that are nonfluorescent in their inactive state but become fluorescent upon activation by enzymatic activity of the target. None of these probes have become available for clinical use, but good results were obtained in in vivo studies of several cancer types [61, 72, 73].
Mucin1
Cell surface associated mucin 1 (MUC1) is a transmembrane glycoprotein that is overexpressed in several types of cancer. Physiologically, mucins are thought to protect the body against infection. However, MUC1 overexpression in cancer is not yet entirely understood. The current idea is that MUC1 protects the cancer cells against the immune system and against chemotherapeutic drugs. Furthermore, it has been shown that MUC1 is able to bind growth factors, which help the tumour cells proliferate [74]. A number of studies have been described using radiolabelled anti-MUC1 antibodies for intraoperative tumour detection and anti-MUC1 antibodies in chemotherapy, reporting beneficial effects. The humanized monoclonal antibody AS1402 is now being studied in a group of patients with recurrent breast cancer and may be an interesting compound for intraoperative imaging when conjugated to a fluorescent agent. Again, this remains speculative, as no experiments in this direction have been reported yet.
Vascular Endothelial Growth Factor
Vascular endothelial growth factor (VEGF) is an important regulator of angiogenesis, playing a role in normal ovarian function as well as in carcinogenesis [75, 76]. Tumour angiogenesis is essential for proliferation, cell survival and dissemination and is promoted through VEGF-related growth factors and receptors. VEGF has several subtypes, of which VEGF-A seems to play the most prominent role in ovarian cancer [77]. By activating two high-affinity tyrosine kinase cell surface receptors, VEGFR-1 and VEGFR-2, intracellular signalling pathways are stimulated leading to endothelial cell recruitment, vascular permeability, proliferation and survival [75].
VEGF is overexpressed in approximately 60–70 % of serous ovarian tumours and is associated with a poor prognosis [78–80]. The anti-VEGF-A monoclonal antibody bevacizumab has shown to improve progression-free survival in both advanced and platinum-resistant ovarian cancer by binding to and neutralizing VEGF-A, preventing its association with endothelial receptors and thus inhibiting angiogenesis [81–84].
Apart from acting as a chemotherapeutical, bevacizumab can be conjugated to radioactive tracers for application in PET imaging, to a fluorescent agent for optical imaging, or both. Radioactive-labelled bevacizumab for detection of tumours has been shown feasible in patients [85, 86]. In a number of animal studies, including intraperitoneal ovarian cancer xenografts, dual-labelled bevacizumab shows similar and persistent uptake in the tumour in both PET and fluorescence imaging [10].
A clinical trial using bevacizumab-IRDye800CW for imaging of VEGF-A-positive breast cancers is currently underway. The next step is to test the value of this fluorescent agent for intraoperative imaging in ovarian cancer. Advantages compared to folate-FITC are that IRDye800CW has excitation and emission wavelengths in the near-infrared range, yielding higher tumour-to-background ratios. A possible disadvantage is the longer distribution time, meaning that the agent needs to be administered approximately 4 days prior to surgery. Furthermore, the specificity in advanced ovarian cancer may be limited by the fact that VEGF is scavenged in fluids, thus also in ascites, which may hamper the detection of small tumour nodules. However, this remains a theoretical problem as preclinical studies report tumour-to-background ratios high enough to detect even submillimeter metastases [10].
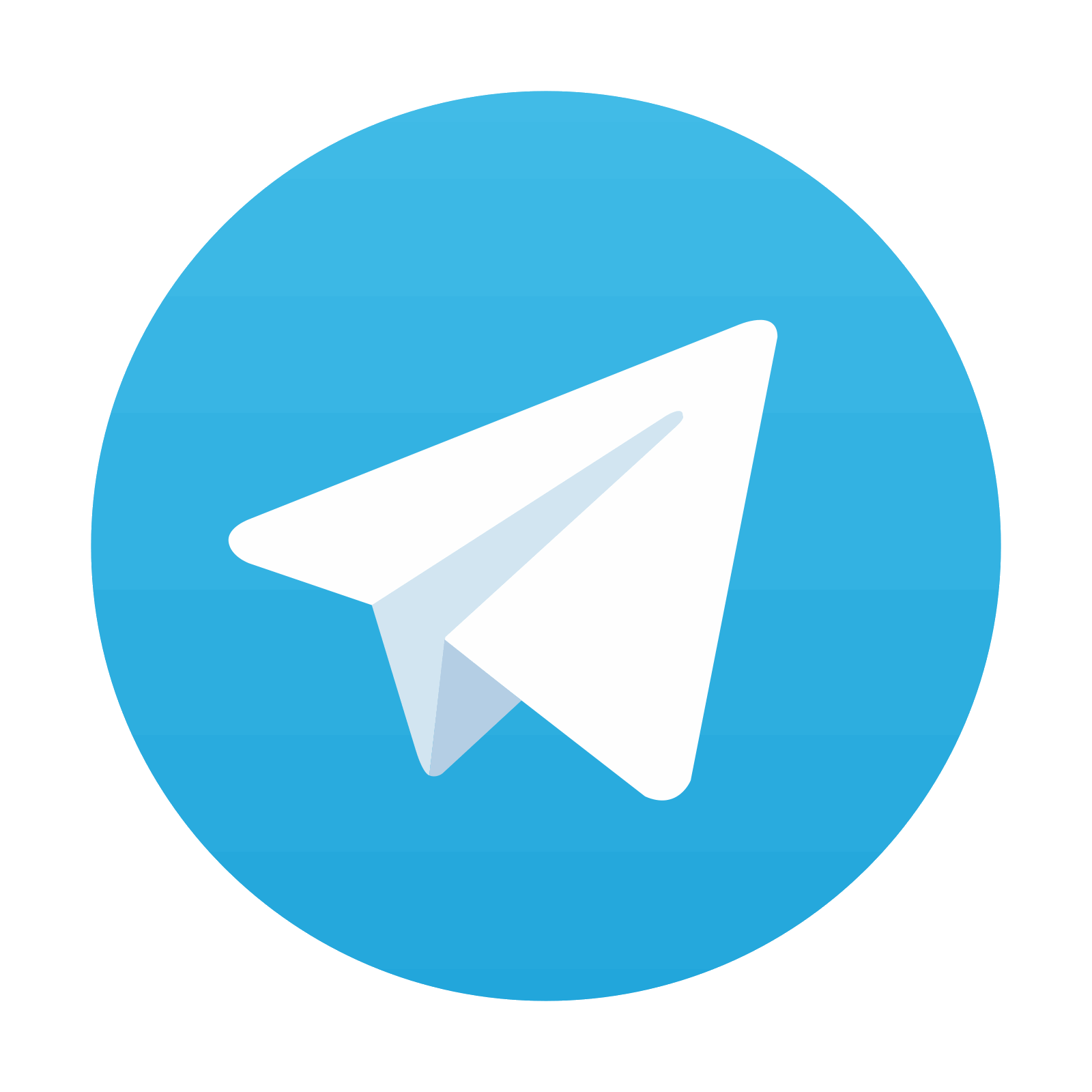
Stay updated, free articles. Join our Telegram channel
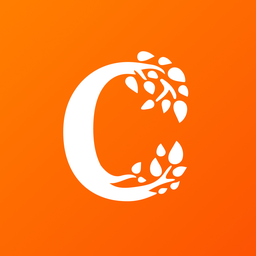
Full access? Get Clinical Tree
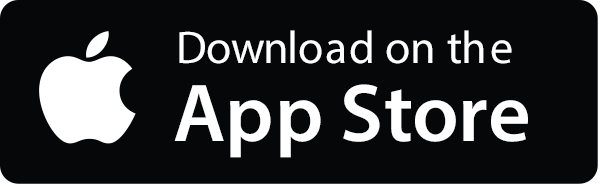
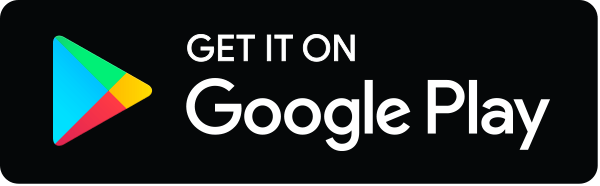