Seth J. Crapp1, Rachel Pevsner Crum2, Nolan Altman2, Jyotsna Kochiyil3, Eshani Sheth3, and Caldon J. Esdaille4 1 Pediatric Teleradiology Partners, Miami, FL, USA 2 Nicklaus Children’s Hospital, Miami, FL, USA 3 Mount Sinai Medical Center, Miami Beach, FL, USA 4 Howard University College of Medicine, Washington, DC, USA In children, as in the adult population, the most common application for 18F‐fluorodeoxyglucose positron emission tomography (18FDG PET‐CT) is whole‐body evaluation for malignancy, staging, and disease extent. The entities most commonly imaged parallel those more commonly encountered in practice, such as Wilm’s tumor, neuroblastoma, lymphoma, and musculoskeletal tumors (Figure 25.1a–c). The unique attributes of PET‐CT allow imaging specialists to analyze the body as an anatomic map of cellular metabolic activity [1, 2]. The degree of cellular metabolism correlates proportionately to the level of radiopharmaceutical activity and can be quantified by maximum standardized uptake value, allowing clinicians to monitor treatment effect, such as necrotic changes or decreasing metabolic activity within a tumor. Hypermetabolic activity not only reflects malignant activity but may be result of other inflammatory or infectious processes (Figure 25.2a–c). Given the wide array of pathologic processes PET‐CT can evaluate, it is critical that technologists and interpreting physicians are given relevant patient history, including recent surgeries, procedures, chemotherapies, and adjunct therapies such as radiation and recent treatment with growth colony stimulating factor. An additional benefit of PET‐CT is evaluation for alternative more easily accessible biopsy sites which can decrease morbidity associated with diagnosis of disease. In asymptomatic patients with rising tumor marker levels PET‐CT plays an important role in detecting occult recurrent malignancy. The range of anatomical coverage on PET‐CT allows for analysis of the entire body with a resolution to detect lesions as small as 4 mm [3]. Positive emission tomography‐magnetic resonance (PET‐MR) fusion combines the superior differentiation of soft tissues and metabolic activity. PET‐MR is advantageous in pediatrics due to decreased dose from ionizing radiation without loss of contrast resolution, which is problematic in low‐dose CT [4, 5]. Functional MRI (fMRI) is an additional application of PET‐MR imaging used in the pediatric population to evaluate epilepsy [6]. An article by myself and Bernal et al. described a post‐processing technique using resting state fMRI (rs‐fMRI) and interictal PET or ictal SPECT images which allows more accurate localization of epileptogenic [7] (Figure 25.3). PET‐CT is also useful in localization of seizure focus [7]. This is performed in the evaluation of patients who have epilepsy refractory to medical management who are considering a surgical resection. PET‐CT is performed interictally. FDG is utilized at a dose of 140 μCi/kg with EEG monitoring to confirm the interictal state. The uptake time is 30 minutes. Epileptogenic regions are usually hypometabolic compared with the normal brain in the interictal period. These studies are most helpful in children where an anatomic lesion is not found on conventional MRI. PET‐MR can be performed on dedicated equipment, but more frequently fusion is done with computer post‐processing image fusion software of the PET‐CT and ‐MR images. There are numerous versions available, but we use Brainlab, which is part of a neuronavigation system that is available to the operating surgeon. This process takes between 30 and 50 minutes. Figure 25.1 (a) 5‐year‐old male with right forehead swelling. Targeted head US shows focal defect (white arrows) in the frontal bone right of midline with complex, fairly circumscribed mass lesion (white asterisk) within the defect. (b) Axial CT image of the brain shows the frontal bone lesion with convex border (white arrows) filling the defect. (c) Sagittal CT of the brain demonstrating frontal bone lesion with mass (white arrow) filling the defect. Figure 25.2 (a) 5‐year‐old male with right forehead swelling. Axial CT of the brain with bone window setting shows frontal bone defect with beveled edges (white arrow) and mass lesion in the defect. (b) Axial 18FDG PET images with attenuation correction of the brain show intense uptake in the area of the frontal bone lesion (white arrows). (c) Fused axial PET‐CT images of the brain demonstrating frontal bone lesion with mass with focal intense radiotracer uptake in the defect (white arrows). The patient was clinically diagnosed with solitary Langerhans cell histiocytosis. The positron‐emitting radiotracer sodium fluoride 18F is used specifically as a bone‐imaging agent in PET imaging. A rise in the use of 18F‐NaF is based on several factors, including superior diagnostic performance over standard technetium‐99m (99mTc)‐based bone scintigraphy, increased availability of PET‐CT in imaging departments, and shortages encountered with 99mTc‐based agents [9]. The unique attributes of 18F‐NaF include rapid clearance from plasma with high retention in the skeleton as well as a high bone‐to‐background ratio in a shorter span of time as compared to standard 99mTc‐based radiotracers, which allows for superior assessment of skeletal trauma [3] (Figure 25.4a,b). Figure 25.3 Example of three patients who underwent evaluation with fMRI for retractable epilepsy (all images are axial in normal radiologic anatomical convention). Case 1: Patient with left temporal lobe cortical dysplasia and refractory complex partial seizures. No evident lesion was found on MRI (top inset). Interictal PET demonstrated a left temporal hypometabolic area in the left temporal lobe (middle inset). The rs‐Mean showed high signal coincident with the PET localization (bottom inset). Case 2: Patient with tuberous sclerosis, presenting with motor seizures characterized by head drops, eyelids twitching, and stiffening of the right arm. Structural MRI showed several cortical–subcortical tubers (top inset). Ictal SPECT showed a left frontal area of slight hyperperfusion (middle inset). The rs‐Mean showed a well‐defined high signal area in the same region, coincident with one of the tubers with high interictal EEG activity (bottom inset). Case 3: Patient with motor seizures consisting of unsteadiness and right facial twitching. Structural MRI showed hyperintensity cortical area on fluid‐attenuated inversion recovery sequences with focal changes compatible with cortical dysplasia in the left frontal region (top inset). Ictal SPET shows two areas of hyperperfusion, one related to the left frontal lesion and a second located in the dorsal right frontal area (middle inset). The rs‐Mean showed a clear cluster of high intensity related to the lesion (bottom inset). (Source: Adapted from Bernal et al. (2015) Utilizing rs‐fMRI’s Mean to Localize Seizure Focus. Open Journal of Radiology, 5, 92–103 [8].) A radiographic skeletal survey is a first‐line requirement in the assessment of child abuse or nonaccidental trauma (NAT), but has some limitations. With the exception of the detection of classic metaphysical lesions (CMLs), 18F‐NaF PET has greater sensitivity in the overall detection of fractures than baseline skeletal survey [10–12]. Figure 25.4 (a) 2‐year‐old male with suspected nonaccidental trauma. Anteroposterior chest radiograph shows no acute or healing fracture. (b) 18F‐NaF 3D Maximum intensity projection (MIP) bone scan showing focal intense radiotracer activity in the left posterior eighth and ninth ribs (black arrows) consistent with acute rib fractures. Radionuclide imaging of the developing pediatric skeleton using 99mTc phosphate compounds has been around since the 1970s and is more routinely used than other bone imaging agents, such as 18F [13]. The role of bone scintigraphy in the evaluation of malignancy has declined due to the advent of PET‐CT but remains a useful diagnostic tool evaluating infection and inflammatory processes such as chronic nonbacterial osteomyelitis (CNO), chronic regional pain syndrome, and osteonecrosis in Legg–Calvé–Perthes disease [14] (Figure 25.5a–c). Familiarity with chronic nonbacterial osteomyelitis is important in the pediatric patient population. Chronic Nonpyogenic Osteomyelitis (CNO) belongs to the autoinflammatory family of rheumatologic disorders related to the absence of sufficient titers of autoantibodies and autoreactive T‐lymphocytes [8]. CNO is a disorder of children and young adults usually diagnosed between 9 and 14 years of age that is characterized by nonbacterial osteomyelitis, usually in multiple sites at the time of diagnosis. Patients present with nonspecific complaints such as pain, tenderness, swelling, or limited range of motion at affected sites. The physical symptoms may also be accompanied by constitutional symptoms such as fever, weight loss, and lethargy less commonly [15]. Initial imaging evaluation is usually done with radiography of the symptomatic site with MRI targeted to the same anatomy if X‐rays are negative. Whole‐body imaging is usually required when CNO is suspected to exclude multiple sites of involvement and is traditionally achieved with 99mTc bone scintigraphy [15, 16]. However, whole‐body MRI is being increasingly used for survey and detection of multifocal osseous lesions [16]. Typically, imaging findings of CNO include lytic and sclerotic lesions affecting the metaphyses of long bones, as well as the medial clavicles. Less common sites of involvement are the vertebrae, pelvic bones, ribs, and the mandible [8, 15, 16]. Since there is currently no diagnostic test available, CNO remains a diagnosis of exclusion. Therefore, it is important for the imager to know specific imaging features as we may be the first to suggest this as a diagnostic possibility. The etiology of proximal femoral epiphysis ischemia or Legg–Calvé–Perthes disease (LCPD) in pediatric patients is related to the occurrence of at least two ischemic episodes. The exact causes of such episodes remain unclear, but are postulated to result from microtrauma, environmental insults, prenatal conditions, genetic predisposition, or hypercoagulable states [17]. Historically, bone scintigraphy was widely used for diagnosis of LCPD, but it is now reserved as a diagnostic tool for a very small number of patients with immense pain or limping (Figure 25.5a–c). Currently, unenhanced MRI has been found to be superior for determining the extent of ischemia in the femoral capital epiphysis [17, 18]. Figure 25.5 (a) 4‐year‐old male with Legg–Calve–Perthes. Initial AP radiograph of both hips shows mild flattening and minor lucency in the right capital femoral epiphysis (CFE). Minor flattening of the left CFE is also observed. (b) AP radiograph of both hips obtained 6 months later shows progression of disease with increased flattening and fragmentation of the right CFE (white arrow) and progressive flattening of the left CFE laterally (black arrow). (c) Planar anterior and posterior images of the hips from 99mTc bone scan obtained at the time of the initial radiograph demonstrate focal photopenia in right CFE (white arrow) consistent with Perthes disease. Low back pain is also a common presenting symptom in pediatric patients, specifically young athletes. The imaging evaluation begins with lumbar spine radiographs, which have limited sensitivity in the detection of pars fractures and stress‐related injuries [19]. Although MRI is the preferred modality if the patient has neurologic symptoms or radicular pain on clinical examination, bone scan with single photon emission computed tomography (SPECT) is superior to MRI and CT in the detection of spondylolysis and remains a critical tool in such cases [19, 20]. Furthermore, bone scan with SPECT‐CT can distinguish fractures from stress reaction, as well as skeletal remodeling, while MRI is superior for evaluation of surrounding soft tissues, intervertebral discs, nerves, and the spinal cord. Neuroblastoma is a malignant neural crest tumor, and the second most common extracranial malignant solid tumor in pediatric patients. Neuroblastoma has the unique capacity to accumulate iodine‐123‐metaiodobenzylguanidine (123I‐MIBG), therefore whole‐body imaging with 123I‐MIBG scintigraphy is performed if a neuroblastoma is strongly suspected clinically and other laboratory tests, such as urinary catecholamines, are positive [21]. Whole‐body 123I‐MIBG scintigraphy is not only important for the diagnosis of neuroblastoma, but also for staging and localization of skeletal lesions (Figure 25.6a–c). 123I‐MIBG follow‐up scans are performed to assess response to therapy. In small number of cases, 123I‐MIBG scintigraphy may be negative at presentation, and PET using 18FDG, 18‐fluoro‐dihydroxyphenylalanine or gallium 68 dodecane tetraacetic acid test‐of‐cure dodecane tetraacetic acid might be indicated. 123I‐MIBG scintigraphy with SPECT‐CT can improve diagnostic accuracy by providing better anatomic localization and may preclude the need for separate diagnostic CT in some cases [21, 22]. Hybrid imaging with CT and SPECT offers the combined benefit of anatomical detail and osteogenic activity, but at an increased cost of higher radiation dose. Pediatric thyroid disease is similar to adult disease and comprises diffuse thyroiditis, thyroid malignancy, and benign nodules. The most common congenital thyroid abnormality is thyroid dysgenesis, total absence of the thyroid gland, which is extremely rare [23]. As pediatric patients are often referred for imaging for palpable abnormalities, ultrasound (US) is the first‐line imaging modality [24]. Nuclear scintigraphy with radioactive iodine‐123 (123I) is usually reserved for patients with hyperthyroid function or diagnosed malignancy prior to evaluation with radioactive iodine‐131 (131I) therapy. Thyroid scintigraphy has a very limited role in the management of Hashimoto thyroiditis (Figure 25.7a–c) and Grave’s disease unless complicated by nodular disease [25, 26]. Thyroid gland hormone synthesis and secretion is regulated by thyroid stimulating hormone (TSH). Iodine is trapped, oxidized, and conjugated in thyroid follicles. Within the follicle, colloid thyroglobulin and peroxidase couple the iodotyrosines, forming thyroxine (T4) and triiodothyronine (T3). Thyroglobulin is only produced by the thyroid gland and is used as a marker for tumor surveillance after radioactive iodine ablation. Radioactive iodine is organified by the thyroid and is ideal to evaluate thyroid function and when combined with US can confirm clinical diagnosis. 123I is preferred over 131I for imaging due to higher energy gamma emission and shorter half‐life. Congenital or acquired decrease in thyroid peroxidase impairs organification of iodine and can be detected by a perchlorate discharge test [27]. 99mTc pertechnetate (99mTc‐NaO4) is administered intravenously and rarely used in our and most pediatric imaging departments. 99mTc‐NaO4 has multiple disadvantages as it is not organified by the thyroid gland, has higher background soft tissue and blood pool activity, and is unreliable for imaging of hypofunctioning nodules, malignant nodules, or ectopic thyroid tissue [25]. In the pediatric population, thyroid nodules are less common but have higher rates of malignancy compared to adults [28, 29]. Initially, palpable thyroid masses should be evaluated by US and correlated with serum TSH. Thyroid scintigraphy should be reserved for evaluation of nodules in hyperthyroid patients only [28, 30]. Nodules in children with normal to elevated TSH (euthyroid/hypothyroid) and suspicious US characteristics such as size >1 cm, solid, irregular margins, hypoechoic, vascular, and microcalcification warrant evaluation with US‐guided fine‐needle aspiration (FNA) [29–32]. Patients with a thyroid nodule and suppressed serum TSH (hyperthyroid) should be further evaluated with 123I thyroid scan and uptake for possible hyperfunctioning autonomous nodule [28] (Figure 25.8a–c). US‐guided FNA may not be necessary unless the hyperfunctioning nodule has a suspicious appearance on US or the patient is asymptomatic [30]. Unlike adults, who are typically treated with 131I ablation, children with toxic nodules are surgically resected due to concern for malignant potential in the adjacent radiosensitive normal tissue [28, 30]. Typically, benign adenomas are hypovascular, echogenic to isoechoic, and have a peripheral hypoechoic halo with peripheral vascularity on sonography. It is important to note that an adenoma cannot be reliably distinguished from follicular carcinoma on imaging and lesions greater than 1 cm will need to undergo FNA to confirm [23, 31, 32]. FNA‐proven benign nodules will require continued serial US follow‐up to assess stability and any new suspicious change. Patients with Hashimoto thyroiditis are also at increased risk for malignancy and nodules with suspicious US features should be sampled with FNA [33–35]. Well differentiated thyroid carcinomas (DTC) are iodine avid and the most common of the thyroid malignancies. Papillary thyroid cancer (PTC) is the most common, occurring in up to 90% of pediatric cases, while follicular thyroid cancer (FTC) is uncommon [26, 28, 30]. PTC usually presents as a nodule but in children can be infiltrative and diffuse. US of the neck should be performed to evaluate for metastatic lymph nodes, which may not be enlarged but will have some distortion of the internal architecture and a similar echotexture to tumor. Adjuvant 131I remnant ablation usually follows primary surgical resection. Post‐treatment 131I whole‐body scans should be performed 5–7 days after treatment due to increased detection of metastatic and residual disease [28]. Surveillance for DTC recurrence includes routine assessment of serum thyroglobulin levels, 123I whole‐body imaging, and annual neck US. 123I whole‐body imaging is best performed with TSH levels elevated to at least 30 μU/mL after either thyroid hormone withdrawal or the use of recombinant human TSH (rhTSH) [28] (Figure 25.9a–c). Figure 25.6 (a) 3‐year‐old male with left adrenal neuroblastoma. Planar anteroposterior and postero‐anterior images from 123I‐MIBG scan show focal intense radiotracer uptake in the left adrenal mass (black arrow), left retroperitoneal lymph nodes (white asterisk) as well as in metastatic foci throughout the skeleton and skull. (b) Sagittal US of the left kidney demonstrating a heterogenous left adrenal mass with stippled calcifications (white arrows). (c) Coronal CT image of the abdomen obtained post therapy showing the complex left neuroblastoma which is inseparable from the upper pole of the left kidney (white arrow) and metastatic left retroperitoneal lymph nodes (black asterisk). Figure 25.7 (a) 14‐year‐old female with Hashimoto’s thyroiditis. US gray‐scale transverse image shows typical appearance of enlarged gland, heterogeneous echotexture, and hypoechoic nodules of infiltrating lymphoid tissue and follicular degeneration. (b) Color Doppler transverse US image shows decreased vascularity. (c) 99mTc scan shows no uptake in the nonfunctioning gland. Markers were placed at the right neck chin and sternal notch. Figure 25.8 (a) 12‐year‐old female with a solitary hyperfunctioning autonomous nodule at the inferior left thyroid lobe. Initial diagnostic FNA of the benign degenerating colloid nodule was confirmed at surgical resection. A large left lobe heterogeneous lesion (arrows) with cystic and solid components is seen on the transverse gray‐scale US image. (b) 99mTc thyroid scintigraphy with pinhole collimator shows a large hyperfunctioning nodule (arrow) at the left lower suppressing the remainder of the gland. Autoimmune thyroiditis is the most common thyroiditis in the pediatric population and is usually diagnosed with thyroid function laboratory tests and US. Hashimoto thyroiditis is the most common cause of pediatric hypothyroidism [26, 33, 34]. Historically, thyroid scintigraphy and perchlorate washout were used in the diagnosis of thyroiditis. Currently, with the advent of US and advanced laboratory tests, thyroid scintigraphy is of little value [25, 36]. If uncontrolled despite antithyroid medication, Graves’ thyrotoxicosis (Figure 25.10a–c) or hashitoxicosis should be evaluated with radioactive iodine uptake and scan, especially if 131I ablation therapy is contemplated [37, 38]. Infectious thyroiditis is uncommon in the pediatric age group and is usually evaluated with US, making thyroid uptake and scan unnecessary. After resolution of infection, CT or MRI is usually performed to evaluate for an underlying infected branchial cleft anomaly or thyroglossal glossal duct cyst. Etiologically, cardiac disease differs in children and adults. In adults, the major cause of ischemia is related to atherosclerotic disease, which is extremely rare in the pediatric patient [39]. Pediatric myocardial perfusion abnormalities are more often related to congenital heart disease (CHD) and anomalies [40, 41]. Perfusion deficits can occur after arterial switch procedure for correction of complete transposition of the great vessels due to stenosis at coronary artery anastomotic sites. SPECT myocardial perfusion imaging (MPI) is an important established test in the assessment of ischemia and scar extent (Figure 25.11). Usually 99mTc‐sestambi myocardial perfusion image testing (MIBI) or 99mTc‐tetrofosmin agents are used in pediatrics due to a short half‐life, decreased absorbed dose, and flexibility of imaging times. In addition, though to a lesser extent than in adults, treadmill exercise can provide added physiologic and aerobic conditioning information. Pharmacologic methods for coronary dilation can also be utilized in infants and very young children who are unable to exercise adequately. Common indications for MPI in the pediatric patient are anomalous coronary artery, postsurgical assessment, and Kawasaki disease (KD). The common disease entities are discussed below. Figure 25.9 (a) 10‐year‐old female with papillary thyroid carcinoma diagnosed by FNA. US gray‐scale image of a large irregular infiltrating hypoechoic mass containing microcalcifications seen at the right lobe (arrows). (b) Chest CT (maximum intensity reconstruction) with numerous pulmonary metastatic nodules. (c) Staging 123I whole‐body scan after total thyroidectomy shows residual and local metastatic lymph nodes in the neck as well as diffuse pulmonary metastases. The patient then received high‐dose radioactive iodine treatment. Figure 25.10 (a) 18‐year‐old female with Graves disease and treated for several years with antithyroid medication (tapazole) but remaining symptomatic and requiring radioactive 131I ablation therapy (12 mCi) for definitive treatment. Diffusely enlarged slightly heterogeneous gland on gray‐scale US. (b) Color Doppler images with typical hypervascularity, termed “thyroid inferno.” (c) Iodine‐123 uptake and scan shows diffusely enlarged gland with lobulated contour. Four‐hour uptake was 59% and 24‐hour was uptake 81%. Figure 25.11 15‐year‐old male patient with history of pulmonary atresia and intact ventricular septum status post Fontaine completion. Myocardial SPECT imaging performed to evaluate chest pain and elevated cardiac enzymes. Partial moderate‐sized scar is present at the inferior and inferior lateral wall junction zone. Also note marked right ventricular uptake of ventricular hypertrophy. Left ventricle ejection fraction 61% with minimal dyskinesia of the scarred wall. The spectrum of hemodynamically significant coronary artery anomalies includes anomalous origin, interarterial course or abnormal connection, congenital fistula, or atresia with varying degrees of symptoms [42, 43]. Coronary anomalies have a reported prevalence of 1–2% in the general population. CT angiography (CTA) is now an accepted standard preferred over conventional angiogram, defining coronary artery anatomy and abnormalities in exquisite detail [43, 44] (Figure 25.12a–c). CTA anatomy should be reviewed in conjunction with interpretation of MPI to better define vessel territory (right/left dominance) and post‐operative anatomy. A left dominant system, present in approximately 10% of the population, can have implications on perfusion of the inferior and septal wall territories [44]. MPI can be helpful in pediatric patients with diagnosed coronary artery anomalies to further assess ischemic symptoms and left ventricle function, as well as aid in pre‐ and postoperative evaluation. Anomalous origin of the left coronary artery from the pulmonary artery (ALCAPA) most often presents in early infancy with massive cardiomegaly due to volume overload and left‐to‐right shunt [39]. Formation of large collaterals between the coronary arteries causes shunting and retrograde flow to the pulmonary artery. Ischemia results from retrograde (steal) blood flow from the myocardium [43]
25
Correlative Imaging of Pediatric Diseases
Positron emission tomography in the pediatric patient
PET‐CT
Neuroimaging with PET‐MRI and PET‐CT
Epilepsy Imaging
18F‐Sodium Fluoride PET
Bone scintigraphy in the pediatric patient
Bone Scan with 99mTc‐labeled Disphosphonates
Chronic Nonpyogenic Osteomyelitis
Legg–Calvé–Perthes Disease
Neurblastoma
Thyroid Scintigraphy in the Pediatric Patient
Pathophysiology
Approach to Pediatric Thyroid Nodules
Thyroid Malignancy
Thyroiditis
SPECT Myocardial Perfusion Imaging in the Pediatric Patient
Coronary Artery Anomalies
Stay updated, free articles. Join our Telegram channel
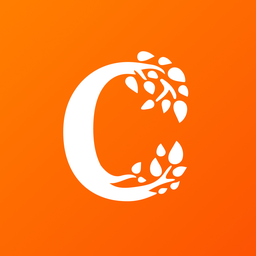
Full access? Get Clinical Tree
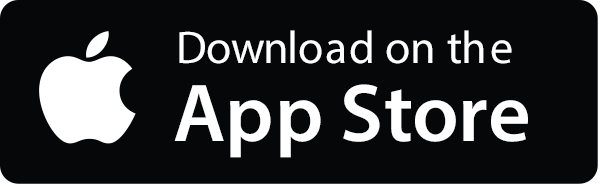
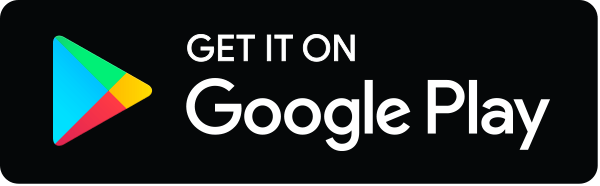