Christopher J. Palestro1,2 and Charito Love3 1 Radiology, Donald & Barbara Zucker School of Medicine at Hofstra/Northwell, Hempstead, NY, USA 2 Nuclear Medicine & Molecular Imaging, Northwell Health, New Hyde Park, NY, USA 3 Radiology, Albert Einstein College of Medicine, Bronx, NY, USA More than 100 years after the discovery of penicillin, infection remains a major threat to mankind. In 2017 more than eight million deaths and 400 000 years of life lost were due to infection, making it first in morbidity and third in mortality among all human diseases [1]. The diagnosis of infection, especially when located deep within the human body, can be challenging and imaging studies are often used for confirmation and localization. Radiological imaging tests, such as X‐rays, ultrasonography, computed tomography (CT), and magnetic resonance imaging, reveal structural alterations in tissues and organs produced by a combination of the infection and the host’s response to the infection. Molecular imaging agents such as gallium, labeled leukocytes, and fluorine‐18 fluorodeoxyglucose (18F‐FDG) reflect the physiological changes that are part of the inflammatory process and can identify abnormalities prior to the appearance of structural changes. The integration of molecular and radiological images with single‐photon emission tomography (SPECT)/CT and positron emission tomography (PET)/CT significantly improves diagnostic confidence and test accuracy. At the present time the most widely used molecular imaging agents for infection are technetium‐99m (99mTc) diphosphonates (for osteomyelitis), gallium‐67 (67Ga) citrate, in vitro labeled leukocytes, and 18F‐FDG. It is important to note that, as useful as these agents are, none of them are specific for infection; they reflect the host response to infection, not the infection itself. 99mTc‐labeled diphosphonates, usually methylene or hydroxymethylene diphosphonate, are used to image the skeletal system. Uptake of these radiopharmaceuticals is dependent on blood flow and rate of new bone formation. When performed for suspected osteomyelitis, a three‐phase bone scan usually is performed. Widely available, relatively inexpensive, and easily performed, bone scintigraphy is extremely sensitive, can become positive within 2 days after the onset of symptoms, and has an accuracy of more than 90% in bones not affected by underlying conditions. Abnormalities on bone scintigraphy reflect the rate of new bone formation in general, not infection specifically, and consequently the test is less useful in the setting of violated bone [2]. 67Ga citrate was one of the first molecular imaging agents used for diagnosing and localizing infection, with initial reports appearing in the early 1970s, and was the mainstay of molecular imaging of infection for many years. Several factors contribute to 67Ga uptake in infection. About 90% of circulating 67Ga is transferrin bound in the plasma. Increased blood flow and vascular membrane permeability result in increased 67Ga delivery and accumulation at infectious foci. 67Ga binds to lactoferrin, which is present in high concentrations in sites of infection. Direct bacterial uptake, complexing with siderophores, and leukocyte transport also contribute uptake of this radiopharmaceutical in infection. Imaging usually is performed 48–72 hours after injection of 185–370 MBq 67Ga. The normal distribution is variable and includes the skeleton, liver, urinary and gastrointestinal tracts, and soft tissues (Figure 26.1) [3]. The use of 67Ga has decreased dramatically over time and presently its use is limited to evaluating pulmonary inflammation and differentiating acute tubular necrosis from interstitial nephritis (Figure 26.2). 67Ga can also be used as a substitute for 18F‐FDG when the latter is not available, for indications such as fever of unknown origin, sarcoid, and spondylodiscitis [4]. Figure 26.1 Normal gallium‐67 scan. There is physiologic distribution of gallium‐67 in the skeleton, liver, spleen, kidneys, and colon on images acquired approximately 48 hours after administration. In the mid‐1970s the demonstration that autologous leukocytes labeled in vitro with indium‐111 (111In) oxine could image infection in humans was a seminal event [5]. In vitro labeled leukocyte (white blood cell [WBC]) imaging rapidly became the molecular imaging test of choice for most infections in the immunocompetent population, and even today remains a valuable diagnostic procedure. The in vitro labeling procedure, which takes about 2–3 hours, can be performed with both 111In oxine and 99mTc exametazime. The usual dosage of 111In WBCs is 10–18.5 MBq; the usual dosage of 99mTc WBCs is 185–370 MBq [6]. WBC uptake depends on intact chemotaxis, number and types of cells labeled, and cellular component of the inflammatory response. A total circulating white blood cell count of at least 2000/μl is needed to obtain satisfactory images. In most clinical settings, the majority of leukocytes labeled are neutrophils, so the procedure is most sensitive for identifying neutrophil‐mediated inflammatory processes, such as bacterial infections. The procedure is less sensitive for infections in which the predominant cellular response is not neutrophilic, such as tuberculosis [6]. Images obtained shortly after WBC reinfusion are characterized by intense pulmonary activity, which clears over time, reaching background levels by about 4 hours. This delayed transit is caused by activation of the cells during labeling, slowing their passage through the pulmonary vascular bed and prolonging their residence in the lungs [7]. The advantages of using 111In as the radiolabel are the stability of the label and a normal distribution of activity limited to the liver, spleen, and bone marrow (Figure 26.3). The 67‐hour half‐life of 111In permits delayed imaging. Patients with musculoskeletal infection may need bone or marrow scintigraphy, which can be performed while the patient’s cells are being labeled, or as simultaneous dual isotope acquisitions, or immediately after completion of the WBC study. Disadvantages include suboptimal photon energies, limited resolution images, and the 18–30‐hour interval between injection and imaging [6]. Figure 26.2 Acute interstitial nephritis. Renal activity is more intense than adjacent lumbar spine activity on these images acquired 48 hours after administration of gallium‐67. The normal distribution of 99mTc WBCs is more variable than that of 111In WBCs because the 99mTc elutes out of the leukocytes. In addition to the reticuloendothelial system, activity is normally present in the urinary tract, large bowel (by 4 hours after reinfusion), and occasionally the gall bladder (Figure 26.4). Imaging usually is performed within a few hours after 99mTc WBC reinfusion. Advantages of 99mTc WBCs include an optimal photon energy for gamma camera imaging, a high photon flux, and the ability to detect abnormalities within a few hours after reinfusion of labeled leukocytes. Disadvantages include urinary tract and bowel activity. The short half‐life of 99mTc is a disadvantage when delayed imaging is needed. When bone or marrow imaging is necessary, an interval of 48–72 hours is required between the WBC and bone or marrow scans [6]. Figure 26.3 Normal indium‐111 labeled leukocyte scan. There is physiologic distribution of labeled leukocytes in the liver, spleen, and bone marrow on images acquired approximately 24 hours after administration. Splenic activity normally is more intense than liver activity. Figure 26.4 Normal technetium‐99m labeled leukocyte scan. In addition to physiologic distribution of labeled leukocytes in the liver, spleen, and bone marrow, there is activity in the urinary tract and large bowel, due to elution of technetium‐99m from the leukocytes. Cellular uptake of 18F‐FDG is governed by three mechanisms: passive diffusion, active transport by an Na1‐dependent glucose transporter (GLUT), and via GLUT‐1 through GLUT‐13 transporters. It is trapped intracellularly but is not metabolized and does not diffuse back into the extracellular space [8]. Uptake of 18F‐FDG in inflammation and infection is related to the effects of the inflammation on inflammatory cells such as neutrophils, lymphocytes, monocytes, and macrophages, which are activated. The number and expression of GLUTs as well as their affinity for deoxyglucose are all increased in activated inflammatory cells [9]. The normal distribution of 18F‐FDG includes the brain, myocardium, and urinary tract. Thymic uptake, especially in children, has been observed. Gastric and bowel activity are variable. Liver, spleen, and bone marrow uptake generally are low grade (Figure 26.5). The FDG molecule is small and enters poorly perfused areas rapidly, so imaging can be performed within 1–2 hours after administration. Skeletal uptake usually normalizes within 3–4 months after trauma or surgery and degenerative bone changes ordinarily show only mildly increased uptake, which are advantages when musculoskeletal infection is a concern [10]. Figure 26.5 Normal 18F‐FDG maximum intensity projection image. There is uptake in the brain, myocardium, liver, spleen, urinary tract, and bowel. Faint bone marrow uptake is also present. The term cardiovascular infection encompasses a wide range of infections, including endocarditis (native and prosthetic valve), cardiac implantable electronic device (CIED), and prosthetic vascular graft infections. Diagnosis of these often life‐threatening conditions can be challenging and imaging plays an important role in their workup. CT and echocardiography detect infection‐induced structural alterations such as vegetations, edema, and abscesses, which typically manifest late in the disease course. Molecular imaging identifies functional changes that accompany infection and potentially can detect these infections before morphological changes develop. Although their uptake mechanisms are different, WBC imaging and 18F‐FDG are both powerful tools for detecting cardiovascular infections. The advent of SPECT/CT and PET/CT has further enhanced their value by improving image quality and providing precise localization of radiopharmaceutical uptake, which is especially important when evaluating relatively small structures like heart valves. Infective endocarditis (IE) is a life‐threatening infection. Despite advances in diagnosis and treatment, patients with IE still have high morbidity and mortality rates. Rheumatic heart disease, mitral valve prolapse, and congenital heart disease, along with prosthetic valves and implantable cardiac devices are all risk factors. These infections begin with the development of a nonbacterial thrombotic endocarditis on the surface of a cardiac valve or other site of endothelial damage, which leads to deposition of platelets and fibrin on the endothelial surface. In a patient with bacteremia, the adherence of circulating bacteria to these sites eventually results in IE [11]. Diagnosis is based on the modified Duke criteria that stratify patients with suspected IE into three categories: definite, possible, and rejected IE, with an overall sensitivity of about 80% [12, 13]. In some instances, the results of blood cultures or echocardiography are inconclusive, thus leading to a high proportion of unconfirmed cases of suspected IE. In one investigation, up to 24% of the patients with pathologically proven IE were classified as possible IE [14]. WBC SPECT/CT is a useful adjunct in patients with suspected IE when echocardiographic, laboratory, and clinical data are contradictory or inconclusive, and when involvement of valves, especially prosthetic valves, needs to be excluded during febrile episodes, sepsis, or postoperative infections (Figure 26.6). In one large investigation, WBC SPECT/CT was 90% sensitive and 100% specific for IE, and was especially useful in patients who were classified as possible IE by the Duke criteria [13]. Figure 26.6 Infected prosthetic aortic valve. There is an area of increased activity (arrow) extending obliquely through the mediastinum on the indium‐111 labeled leukocyte maximum intensity projection image (a). On the coronal SPECT/CT image (b) the abnormal activity (arrows) involves the prosthetic valve and extends into the aorta. Transthoracic echocardiography (not shown) was negative. The consequences of IE are not limited to the heart. Parts of the vegetation may separate from the infected valve and travel through the bloodstream. These septic emboli can cause embolic strokes and infarctions of the spleen, kidneys, and mesentery [12]. Performing whole‐body WBC imaging as well as WBC SPECT/CT of the chest can identify remote sites of infection in patients with as well as those without IE, thereby guiding patient management [13]. 18F‐FDG also is useful for diagnosing IE with a pooled sensitivity and specificity of 61% and 88%, respectively (Figure 26.7). Despite the relatively low overall sensitivity, 18F‐FDG is useful in patients with prosthetic cardiac valves and is more sensitive but less specific than WBC imaging in this population. False‐negative results have been attributed to small lesions below the limits of resolution of current systems and antibiotic treatment for more than 1 week prior to imaging. False‐positive results are associated with postoperative inflammation and the presence of severe prosthetic valve thrombosis. 18F‐FDG and WBC SPECT/CT are complimentary procedures; it has been suggested that WBC imaging be reserved for those cases in which 18F‐FDG is inconclusive and during the first 2 months after cardiac surgery [15, 16]. CIEDs, which include permanent pacemakers, implantable cardioverter‐defibrillators, and cardiac resynchronization systems, have become increasingly important in the management of cardiac disease, significantly improving patients’ quality and quantity of life. The number of devices implanted has increased dramatically over the past several years, as has the number implanted in older patients with more comorbidities, which has led to higher rates of infection. Risk factors for CIED infection include diabetes, renal failure, corticosteroid and oral anticoagulation use, coexisting patient morbidities, periprocedural factors such as failure to administer perioperative antimicrobial prophylaxis, amount of indwelling hardware, revision procedure, physician experience, and microbiology of bloodstream infection [17]. Figure 26.7 Infected prosthetic mitral valve. There is a circumferential area of intense 18F‐FDG uptake surrounding the prosthetic mitral valve. Molecular imaging is especially useful in patients with prosthetic heart valves. Staphylococcal species account for 60–80% of CIED infections. The pocket of a CIED may become infected at the time of implantation, during subsequent surgical manipulation of the pocket, or if the generator or subcutaneous electrodes erode through the skin. Pocket infections can spread along the intravascular portion of the electrode, extending into the intracardiac portion of the device. The pocket or intracardiac portion of the electrode also may become infected secondary to hematogenous spread of infection from a distant site [17]. Most CIED infections are accompanied by local inflammatory changes of the generator‐pocket site, or cutaneous erosion with percutaneous exposure of the generator and/or leads. Transesophageal echocardiography is useful for identifying CIED‐related endocarditis in adults. Cultures of generator‐pocket‐site and lead tips confirm the diagnosis and identify the causative organism [17]. WBC SPECT/CT is useful for confirming the presence of CIED‐associated infection, defining the location and extent of infection, and detecting associated complications (Figure 26.8). A negative study excludes CIED infection during febrile episodes and sepsis. Performing whole‐body imaging in addition to SPECT/CT facilitates the identification of remote sites of infection and identifies alternative causes of infection in patients without CIED infection [18–20]. 18F‐FDG also is useful for diagnosing CIED infections (Figure 26.9). In addition to diagnosing pacemaker pocket infection, 18F‐FDG delineates the extent of infection and can facilitate the differentiation of infection from post‐implantation changes. 18F‐FDG improves the diagnostic accuracy of the modified Duke criteria for CIED infection and is useful for making an early and accurate diagnosis of left ventricular assist device infection, determining the extent of infection, and monitoring response to therapy [21–24]. In a meta‐analysis that included nearly 500 patients, the pooled sensitivity of 18F‐FDG PET/CT for diagnosing CIED infection was 83%. The pooled specificity was 89%. For diagnosing pocket infection, pooled sensitivity and specificity were 96 and 97%, respectively. The test was less sensitive for lead infection and CIED‐IE with pooled sensitivity and specificity of 76 and 83%, respectively [25]. False‐negative 18F‐FDG results in CIED infections are associated with lesions below the limits of resolution of currently available systems and previous antibiotic treatment, especially for more than 1 week. False‐positive results are caused by postoperative inflammation and severe prosthetic valve thrombosis [21]. Figure 26.8 Pacemaker pocket infection. There is intense indium‐111 labeled leukocyte accumulation in the left upper chest, which, as seen on the coronal SPECT image, is confined to the surgical pocket. There are no other abnormalities on the whole‐body image, which excludes remote sites of infection. Figure 26.9 Pacemaker pocket infection. There is intense 18F‐FDG accumulation in the left upper chest surrounding the pacemaker pocket (arrows) (Source: Courtesy Dr. G. Abikhzer.) In a metanalysis comparing 18F‐FDG PET/CT and WBC SPECT/CT in CIED infections the pooled sensitivity and specificity of 18F‐FDG PET‐CT were 87% and 94%, respectively. There were not enough data to perform a metanalysis for WBC SPECT/CT, but in the two studies included, sensitivity exceeded 90% and specificity was 100% [26]. Although infrequent, prosthetic vascular graft infection is associated with a high morbidity and, in some situations, mortality. Underlying comorbidities, such as diabetes mellitus and compromised immunity, increase risk of infection and infection‐related complications, such as sepsis, enteric fistulae, spread of infection to other sites, and death. Prosthetic vascular grafts in the groin or lower extremities are extracavitary grafts, while those in the abdomen and thorax are intracavitary grafts [27]. The most common cause of vascular graft infection is intraoperative bacterial contamination. The second most common cause is spread of infection from a contiguous site. Other causes include bacterial colonization of a thrombus or direct inoculation of infection, and hematogenous spread from a remote infection [27]. The clinical presentation of vascular graft infection depends on the graft location, pathogenesis of the infection, and the time since surgery. In early (within 2 months after surgery) extracavitary graft infections patients have fever, chills, leukocytosis, and other findings of sepsis. Physical findings include wound erythema, abscess, sinus tract drainage, peripheral septic emboli, anastomotic rupture with hemorrhage, and erosion of the graft through the wound. Patients with late infections, those that develop more than 2 months postoperatively, have a more indolent presentation, characterized by groin erythema, painful swelling, sinus tract drainage, and erosion of the graft through the skin. Frank systemic signs of sepsis usually are absent [27]. Abdominal vascular graft infections develop months to years after graft placement, usually without obvious physical findings. Symptomatic patients may have abdominal pain, fever, leukocytosis, sepsis, and, when there is erosion into the bowel, gastrointestinal bleeding [27]. Thoracic infections involving the aortic root can have a presentation like that of IE: fever, chills, heart failure, and disruption of the anastomotic suture line of the aortic root. Sepsis is common. Infections associated with repair of an aortic aneurysm or aortic dissection usually occur within 3 months postoperatively. Septic emboli can occur peripherally as well as in the central nervous system. Anastomotic rupture can result in sudden massive, often fatal, hemorrhage [27]. WBC imaging has been used for diagnosing prosthetic vascular graft infection for many years. The sensitivity varies from 73% to 100% and is not affected by antibiotic therapy or duration of symptoms. Specificity ranges from about 80% to 100% [28–32]. False‐positive results can occur with thrombosed grafts, bleeding, perigraft hematomas, pseudoaneurysms, lymphoceles, and uninfected grafts less than 1 month old [6]. Based on available data, the test is more accurate for extracavitary than intracavitary graft infections. SPECT/CT improves test accuracy (Figure 26.10) [33, 34]. In one investigation SPECT/CT was more sensitive (100% vs. 81.5%) and more specific (100% vs. 62.5%) than SPECT alone and was especially useful for detecting, localizing, and defining the extent of infection [34]. 18F‐FDG accurately diagnoses prosthetic vascular graft infection, with sensitivity and specificity ranging from 88% to 100% [35, 36]. Prosthetic vascular grafts, like any other foreign material placed into a person’s body, can incite a foreign‐body inflammatory reaction. This inflammatory reaction can be associated with increased 18F‐FDG activity, even in the absence of infection. Knowledge of typical 18F‐FDG uptake patterns associated with infection and foreign body reaction improves the accuracy of the test. Vascular graft infection generally presents as focal or heterogeneously increased 18F‐FDG uptake that projects over the vessel on the CT component of the examination (Figure 26.11). The aseptic foreign body reaction appears as linear, diffuse, homogeneous uptake along the graft (Figure 26.12) [37, 38]. The patched pattern of 18F‐FDG uptake, seen in patients in whom adhesives are used to secure the graft, is not indicative of infection [39]. Figure 26.10 Infected aortic endovascular stent. On the anterior planar indium‐111 labeled leukocyte image (a) there is an area of increased activity overlying the mid lumbar spine (arrowhead). On the coronal SPECT/CT image (b) this activity involves the stent (arrows). Recent data suggest that WBC SPECT/CT is superior to 18F‐FDG for diagnosing prosthetic vascular graft infection. In a metanalysis the pooled sensitivities of 18F‐FDG PET, 18F‐FDG PET/CT, WBC imaging, and WBC SPECT/CT were 94%, 95%, 90%, and 99%, respectively. The pooled specificities were 70%, 80%, 88%, and 82% respectively. Pre‐ and post‐test results showed that WBC SPECT/CT with a positive post‐test probability of 96% is superior to 18F‐FDG PET/CT with a positive post‐test probability of 83% [40]. In a prospective investigation, 39 patients with 96 prosthetic vascular grafts who were suspected of having prosthetic vascular graft infection underwent WBC SPECT/CT and 18F‐FDG PET/CT. Nineteen of the 96 grafts were infected. The sensitivity, specificity, and accuracy of 18F‐FDG PET/CT were 85%, 68.4%, and 71.9%, respectively. The sensitivity, specificity, and accuracy of the WBC SPECT/CT scans were 89.5%, 90.9%, and 90.6%, respectively. Interobserver agreement was good for 18F‐FDG PET/CT (kappa value of 0.76) and excellent for WBC SPECT/CT (kappa value of 0.97) [41]. Figure 26.11 Infected aortic graft. There is intense heterogeneous 18F‐FDG uptake in this abdominal aortic graft (arrow). Monitoring treatment response is critical to the successful management of infectious diseases. There is a growing body of evidence that suggests 18F‐FDG is useful for monitoring treatment response and can provide prognostic information in patients with prosthetic vascular graft infection. In one investigation 18F‐FDG PET/CT had an impact on patient management in all 25 patients with infected prosthetic vascular grafts. Antibiotic therapy was continued in 19 patients (76%), changed in four (16%), and discontinued in two (8%). 18F‐FDG PET/CT identified additional abnormalities, which further impacted patient management, in eight patients [42]. The results of the prospective Vascular Graft Infection Cohort Study suggest that consecutive 18F‐FDG PET/CTs could influence clinical decision‐making in patients with aortic graft infection [43]. Figure 26.12 Uninfected aortic graft. There is faint, homogeneous 18F‐FDG uptake in the graft, best appreciated on the coronal PET image (arrows). Compare the uptake in this graft with the uptake in the infected graft in Figure 26.11. Osteomyelitis, which is an infectious process of the bone, is caused by bacteria, viruses, and fungi. The infection may be localized or may involve periosteum, cortex, marrow, and cancellous tissue and can arise either hematogenously or via direct or contiguous inoculation. Acute hematogenous osteomyelitis is caused by seeding of organisms transported through the bloodstream from a remote source to the bone and occurs most often in children. The highly vascular metaphyses are the most commonly affected parts of the bone, presumably because slowed blood flow predisposes the metaphyseal vessels to thrombosis and the bone itself to localized necrosis and infection [44]. Acute osteomyelitis can also be due to the spread of organisms from direct trauma, a contiguous focus of infection, and postoperative sepsis. Conditions that predispose to osteomyelitis include diabetes mellitus, sickle cell disease, intravenous drug abuse, alcoholism, and immunosuppression. Open fractures, recent orthopedic surgery, and joint prostheses also are risk factors for osteomyelitis [44]. Imaging tests are performed routinely as part of the diagnostic workup. One of the most widely used molecular imaging tests for diagnosing osteomyelitis is three‐phase bone scan scintigraphy, which consists of a dynamic imaging sequence, the flow or perfusion phase, followed immediately by static images of the region of interest, the blood pool or soft tissue phase. The third, or skeletal, phase consists of images of the area of interest acquired 2–4 hours after injection. Focal hyperperfusion, focal hyperemia, and focally increased bony uptake are the classic presentation of osteomyelitis on a three‐phase bone scan. Widely available, relatively inexpensive, and easily performed, bone scintigraphy is extremely sensitive and can become positive within 2 days after the onset of symptoms. The test is both sensitive and specific for diagnosing osteomyelitis in bones not affected by underlying conditions. In a review of 574 patients, the sensitivity and specificity of three‐phase bone scintigraphy were 94% and 95%, respectively [45]. Unfortunately, abnormalities on bone scintigraphy reflect the rate of new bone formation in general, not infection specifically. In the setting of preexisting conditions such as fracture, orthopedic hardware, and degenerative joint disease, because of increased bony remodeling, the specificity decreases (Figure 26.13). In a review of 592 patients with underlying bony abnormalities, the sensitivity of the three‐phase bone scan was 95%, while the specificity was only 33% [45]. Thus, in patients with preexisting conditions such as fractures and orthopedic hardware, bone scintigraphy is of limited value. Figure 26.13 Left tibial fracture nonunion. On the radiograph (a) performed 6 months after intramedullary rod fixation of left tibial midshaft fractures, there is minimal healing. The three‐phase bone scan (b) demonstrates focal hyperperfusion, focal hyperemia, and focally increased activity in the fracture site, the classic presentation of osteomyelitis. There was no evidence of infection at surgery. In the setting of underlying osseous abnormalities, the bone scan is sensitive but not specific. (Source: Reprinted with permission from Love C, Palestro CJ. Nuclear medicine imaging of bone infections. Clin Radiol. 2016;71(7):632–46.) No one agent is equally efficacious in all regions of the skeleton. The selection of the appropriate study is based on the clinical question posed. In adults it is helpful to divide musculoskeletal infections into three general categories: periprosthetic joint infection, spondylodiscitis, and diabetic foot osteomyelitis. Periprosthetic joint infections are infections that involve the joint prosthesis and adjacent tissues. These infections are an infrequent complication of lower extremity prosthetic joint surgery. The prevalence of periprosthetic joint infection up to 2 years following hip replacement is 1.63% and following knee replacement is 1.55%. Both procedures have a prevalence of more than 2% at 10 years. The incidence and the prevalence of these infections are increasing because of the increasing number of these procedures being performed and the increased life expectancy of patients undergoing these procedures. Approximately one‐third of periprosthetic joint infections develop within 3 months (early), another third within 1 year (delayed), and the remainder more than 1 year (late) after surgery. Early and delayed infections are thought to be caused by organisms introduced at surgery; late infections are more likely due to hematogenous spread of infection [46–50]. Diagnosing periprosthetic joint infection and differentiating it from other causes of prosthetic joint failure is extremely important because although many causes of prosthetic failure can be treated with single‐stage exchange arthroplasty during one hospital admission with one surgical intervention, the treatment of periprosthetic joint infection is more complicated. An excisional arthroplasty is performed followed by weeks to months of antibiotic treatment and eventually a revision arthroplasty. A sensitive but nonspecific test can lead to multiple costly operations when a single intervention would have sufficed. A specific, but insensitive, test will result in additional surgical interventions because undiagnosed infection will cause any revision implant to fail with potentially serious consequences [51]. Pain, the most common symptom associated with periprosthetic joint infection, is present in 90–100% of cases. The presence of fever is variable, ranging from less than 5% to more than 40% of patients with infection. Erythema and joint swelling are often present in acute infections but are less common in chronic infections. Leukocytosis is a poor predictor of infection. The erythrocyte sedimentation rate peaks during the first postoperative week and can remain elevated for up to a year, while the C‐reactive protein level can remain elevated for up to 3 weeks after uncomplicated arthroplasty. Joint aspiration with culture is the definitive preoperative diagnostic procedure for periprosthetic joint infection. Although specific, the sensitivity is variable [52]. Molecular imaging is extremely useful in the evaluation of periprosthetic joint infection. Bone scintigraphy often is the initial molecular imaging test performed. A normal study makes infection unlikely, while an abnormal study requires further investigation. In a systematic review, the pooled sensitivity and specificity of bone scintigraphy for diagnosing periprosthetic hip infection were 80% and 69%, respectively. The specificity was less than that of labeled leukocyte scintigraphy (77%), combined labeled leukocyte/bone marrow scintigraphy (95%), and 18F‐FDG (84%). Two studies included in the systematic review evaluated bone scintigraphy with SPECT. The sensitivity was 100% in both studies and the specificity was 65% and 68% [53]. In a metanalysis of periprosthetic joint infection of the knee, the pooled sensitivity and specificity of bone scintigraphy were 93% and 56%, respectively. Two studies included in the systematic review evaluated bone scintigraphy with SPECT. The sensitivity was 100% in both studies and the specificity was 65% and 68% [54]. Performing bone scintigraphy as a three‐phase bone scan has not improved the accuracy of the test [55]. Limited data suggest that bone scintigraphy with SPECT/CT is better than planar bone imaging for diagnosing periprosthetic joint infection, but comparative studies with other molecular imaging techniques are lacking [56, 57]. 67Ga imaging, alone and in combination with bone scintigraphy, has also been used to diagnose lower extremity periprosthetic joint infection. The sensitivity and specificity of 67Ga scintigraphy for periprosthetic hip joint infection range from 37% to 83% and from 77% to 100%, respectively [55]. In a meta‐analysis the sensitivity and specificity of combined bone and 67Ga scintigraphy for diagnosing of periprosthetic hip joint infection were 59% and 97%, respectively [53]. The test offers little improvement over bone scintigraphy alone and with the availability of labeled leukocytes and, more recently, 18F‐FDG, there is no role for 67Ga in the diagnostic workup of periprosthetic joint infection. For many years combined WBC/bone marrow scintigraphy has been the molecular imaging test of choice for diagnosing lower extremity periprosthetic joint infection. In a meta‐analysis, the pooled sensitivity and specificity of combined WBC/bone marrow scintigraphy for diagnosing periprosthetic hip joint infection were 69% and 96%, respectively [53]. In a meta‐analysis, the pooled sensitivity and specificity of combined WBC/bone marrow scintigraphy for diagnosing periprosthetic knee joint infection were 80% and 93%, respectively (Figure 26.14) [54]. The role of 18F‐FDG PET for diagnosing periprosthetic infection of the hip was reviewed in three meta‐analyses [53, 58, 59]. In one meta‐analysis, the pooled sensitivity and specificity of 18F‐FDG‐PET were 86% and 93%, respectively. It was significantly more specific than bone scintigraphy and significantly more sensitive than combined WBC/bone marrow scintigraphy [53]. In a second meta‐analysis, pooled sensitivity and specificity were both 88% [58]. In the third meta‐analysis, pooled sensitivity and specificity of 18F‐FDG‐PET were 83% and 90%, respectively (Figure 26.15) [59]. Three meta‐analyses reviewed the diagnosis of periprosthetic knee infection with 18F‐FDG [54, 58, 59]. In one meta‐analysis the pooled sensitivity and specificity were 70% and 84%, respectively [54]. In another, pooled sensitivity and specificity were 72% and 80% respectively [58]. In the third meta‐analysis, pooled sensitivity and specificity were 90% and 75%, respectively [59]. Figure 26.14 Periprosthetic infection right knee. On the planar images (a) there is a subtle mismatch on the labeled leukocyte and bone marrow images (arrows). On the dual isotope SPECT/CT coronal images (b), the mismatch is much more obvious (arrows). Figure 26.15 Periprosthetic infection right hip. There is a large area of 18F‐FDG accumulation along the lateral aspect of the right hip arthroplasty, best delineated on the fused image (arrow) (Source: Courtesy Dr. G. Abikhzer.) In summary, combined WBC/bone marrow scintigraphy and 18F‐FDG are the most appropriate molecular imaging tests for diagnosing periprosthetic joint infection of the hip and knee. 18F‐FDG is the most sensitive, while combined WBC/bone marrow scintigraphy is the most specific test. Bone scintigraphy is a sensitive imaging technique but lacks the specificity to differentiate among the various conditions that cause painful hip and knee prostheses. It is a good rule‐out test, but not a good rule‐in test. Shoulder joint replacement surgery was first performed in the United States in the 1950s to treat severe shoulder fractures, and over the years this procedure has come to be useful for a variety of painful shoulder conditions [60]. Periprosthetic joint infection is a serious complication of shoulder arthroplasty, with a reported incidence between 1% and 10%. These infections are classified as early (≤3 months), delayed (3–24 months), and late (>24 months) and can be further classified, based on duration of symptoms, as acute (<6 weeks) or chronic (>6 weeks). Acute infection presents as septic arthritis with erythema, swelling, pain, and abnormal laboratory test results and is readily diagnosed. Diagnosing chronic infection is more challenging and arthrocentesis is required for definitive diagnosis [61]. Data on molecular imaging in periprosthetic shoulder arthroplasty infection are limited. On bone scintigraphy the normal evolution of periprosthetic uptake around the various types of shoulder arthroplasties has not been established and there are no data on the role of bone scintigraphy, alone or in combination with 67Ga, for diagnosing periprosthetic shoulder joint infection. In one investigation combined WBC/bone marrow scintigraphy with SPECT/CT was 18% sensitive (2/11) and 100% specific (18/18) [62]. In another investigation, the sensitivity and specificity of 18F‐FDG PET/CT were 14% and 91%, respectively [63]. With such limited data it is difficult to make definitive recommendations about the role of molecular imaging in the diagnosis of periprosthetic shoulder joint infection. At the present time it appears that both combined WBC/marrow imaging and 18F‐FDG PET/CT, because of high specificity, are good rule‐in tests, i.e. when they are positive there is a high likelihood of infection. A negative result, however, does not exclude infection. Spondylodiscitis is an infection of the vertebral body and/or disc and accounts for about 1% of all cases of osteomyelitis. The infection may extend into the epidural space, posterior elements, and paraspinal soft tissues. The lumbar, thoracic, and cervical spine, in decreasing order of prevalence, are the major sites of involvement. In about 65% of cases, the infection is limited to a single spinal segment: two contiguous vertebral bodies and the intervening disc. Multilevel contiguous infection occurs in about 20% of cases and noncontiguous infection in about 10% of cases. Predisposing risk factors include advanced age, diabetes mellitus, coronary artery disease, spinal interventions, immunosuppression, intravenous drug use, and comorbidities such as endocarditis and urinary tract infection. Staphylococcus aureus (60%) followed by Enterobacter species (30%) are the most common offending organisms [64]. In developing countries and among HIV‐infected patients, Mycobacterium tuberculosis is an important cause of spondylodiscitis, affecting the thoracic spine more frequently than the rest of the spine and with a propensity for multilevel involvement [65]. Approximately 95% of pyogenic cases of spondylodiscitis involve the vertebral body, with only 5% involving the posterior elements. Posterior element involvement is more frequent in tuberculous and fungal than in pyogenic infections [64]. Spondylodiscitis frequently is an indolent disease. There may be a long interval between onset of symptoms and diagnosis [66]. Back pain, which is present in a myriad of other conditions affecting the spine, followed by fever are the most common presenting symptoms. C‐reactive protein levels and erythrocyte sedimentation rate can be elevated, but are not specific, and the peripheral white blood cell count is not sensitive [64]. Bone scintigraphy frequently is used as a screening test in suspected spondylodiscitis. However, false‐negative results have been reported in elderly individuals, presumably due to atherosclerosis‐induced ischemia. In addition, bone scintigraphy is not useful for detecting the soft tissue infections that often accompany, or mimic, spondylodiscitis. The bone scan can remain abnormal for some time after the infection has resolved due to ongoing bony remodeling during healing. Performing the test as a three‐phase bone scan does not improve accuracy because although it improves specificity, it does so at the expense of sensitivity [2]. 67Ga, alone and in combination with bone scintigraphy has been used to diagnose spondylodiscitis. 67Ga improves the bone scan specificity, may detect infection sooner than the bone scan, and can identify accompanying soft tissue infections, which frequently go unrecognized on bone scintigraphy [2]. Incorporating SPECT/CT into the 67Ga imaging protocol has incremental value by precisely localizing radiopharmaceutical uptake and improving disease detection (Figure 26.16) [64]. In a retrospective investigation, combined bone/67Ga SPECT/CT detected 17/18 cases of spondylodiscitis and was negative in all 16 patients without infection (97% accuracy) [67]. WBC imaging is not useful for diagnosing spondylodiscitis because, for reasons that are not well understood, 50% or more of all cases present as areas of decreased or absent activity. The photopenic presentation is associated with several conditions in addition to active infection, including treated spondylodiscitis, tumor, infarction, compression fracture, and Paget’s disease [64]. The role of 18F‐FDG in the diagnosis of spondylodiscitis has been extensively investigated and the results have been uniformly excellent. In one meta‐analysis, the pooled sensitivity and specificity of 18F‐FDG PET/PET‐CT were 97% and 88% [68]. In another, more recent, meta‐analysis, 18F‐FDG PET/CT had a pooled sensitivity of 94.8% and a pooled specificity of 91.4% (95% CI, 78.2–96.9%) [69]. 18F‐FDG is superior to single‐photon emitting radiopharmaceuticals for detecting both bone and soft tissue infections and for differentiating severe arthritic changes from infection [64].
26
Infection/Inflammation Imaging
Radiopharmaceuticals
99mTc Diphosphonates
67Ga Citrate
In vitro Labeled Leukocyte Scintigraphy
18F‐fluorodeoxyglucose
Infections
Cardiovascular Infections
Infective Endocarditis
Cardiac Implantable Electronic Device Infections
Prosthetic Vascular Graft Infections
Musculoskeletal Infections
Periprosthetic Joint Infection
Spondylodiscitis
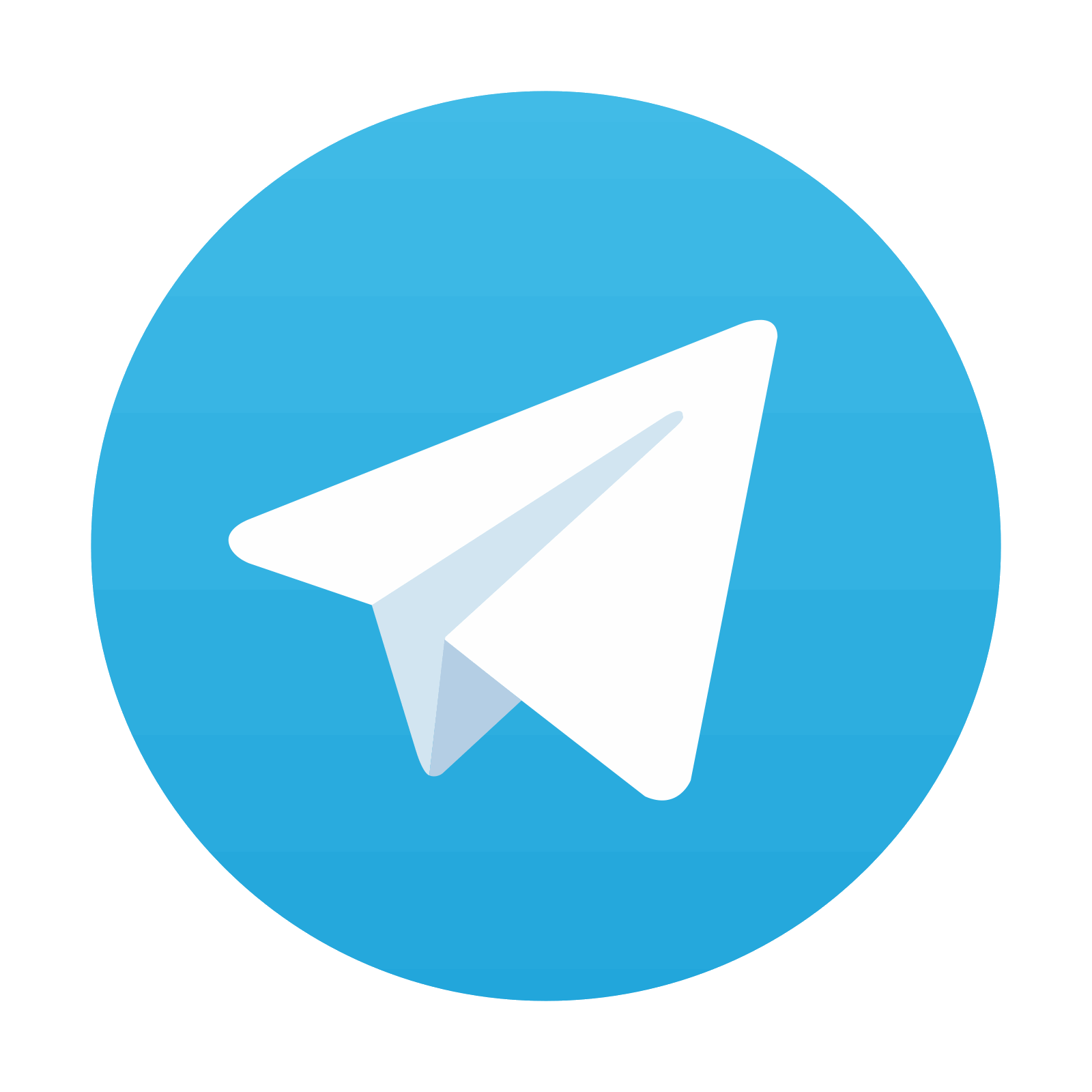
Stay updated, free articles. Join our Telegram channel
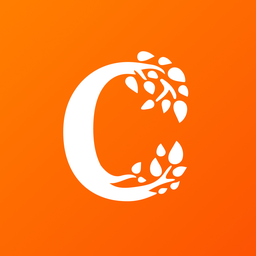
Full access? Get Clinical Tree
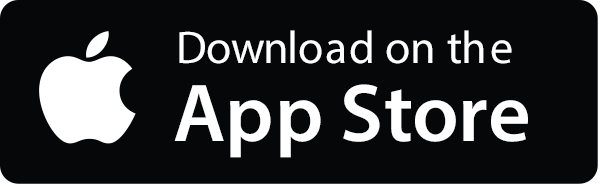
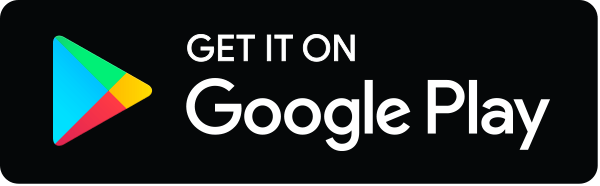