Radionuclide
Half-life (h)
Mode of decay
β+ Particle energy (MeV)a,b
β+-Branching ratio (%)b
Major γ-photons emitted other than annihilation photons in MeV (% abundance)
18F
1.8
β+/ECD
0.633
97.0
None
44Sc
3.9
β+/ECD
1.474
94.3
1.157 (99.9)
64Cu
12.7
β+/β–/ECD
0.653
17.4
1.346 (0.47)
68Ga
1.1
β+/ECD
1.889
88.0
1.077 (3.3)
69Ge
39.1
β+/ECD
1.205
21.0
0.574 (13.3), 0.872 (11.9)
72As
26.0
β+/ECD
2.499
64.2
0.630 (7.9), 0.834 (80.0),
86Y
14.7
β+/ECD
1.220
11.9
0.443 (16.9), 0.646 (9.2), 0.777 (22.4), 1.854 (17.4), 1.920 (20.8)
89Zr
78.4
β+/ECD
0.897
23.0
0.909 (100)
PET images acquired after in vivo administration of radiolabeled nanoplatforms are generally analyzed by defining regions of interest and extracting radioactivity versus time-curves for the region [16, 18]. In more realistic circumstances, functional parametric images are generated on a voxel-to-voxel basis using generic kinetic analysis [18]. If effect of drug-loaded nanoplatforms needs to be quantified, mathematical kinetic modeling approach can be employed [16, 18]. This strategy helps to enhance data interpretation within a framework of important kinetic behavior in the region of interest to obtain quantitative parameters of relevance and universal comprehension. Mathematical modeling of PET data also enables pharmacological and physiological parameters that can be used to quantitatively assess the in vivo behavior of drug-loaded nanoplatforms [16, 18].
3 Radiolabeled Nanoplatforms for PET Image-Guided Drug Delivery
In order to achieve image-guided drug delivery, researchers have developed several nanoplatforms with diverse sizes, architectures, and surface properties for selective administration of the chemotherapeutic drug to a specific target location [11, 12]. Some typical examples of nanoplatforms that have been particularly found to be useful for PET-image-guided drug delivery include organic nanoparticles such as liposomes, micelles, endogenous nanostructures, and inorganic nanoparticles such as colloidal metals and oxide nanoparticles [11, 26]. For a particular application, the choice of the nanoplatform is influenced by the bioavailability, biodistribution, types of drugs that can be delivered, and the specificity and pharmacokinetics of delivery. The in vivo stability and fate of the drug-loaded nanoplatforms is decided by numerous factors such as size, rigidity, charge, solubility, and surface modifications of the nanoplatform. With the advances in material science and nanotechnology, it is now possible to specifically tailor these properties during the synthesis of nanoplatforms for targeted delivery of requisite doses of chemotherapeutic drugs and imaging contrast agents into cancerous lesions while sparing the healthy tissues. Such “smart” theranostic nanoplatforms hold out the possibility of radically changing the practice of cancer management, allowing easy diagnosis followed by effective targeted therapy at the early stages of the disease.
Generally, two strategies are used for loading drugs onto targeting nanoplatforms [11, 12]. In the first approach, drugs are directly conjugated to the nanoplatforms surface using suitable linkers [11, 12]. The major limitations of this approach include (a) potential alteration in property of the drug due to conjugation, (b) inevitable heterogeneity of the final product, and (c) the need to develop customized conjugation procedure for each particular drug that needs to be delivered using the nanoplatform [11, 12]. Many of these limitations could be circumvented to a considerable extent in the other approach, which involves loading of chemotherapeutic drugs onto high capacity nanoplatforms (e.g., liposomes, inorganic oxides, etc.) [11, 12]. Such drug-loaded nanoplatforms protect entrapped drugs from degradation during their delivery to the target and do not alter the biological efficacy of the drug [11, 12]. Despite excellent attributes of this strategy for PET image-guided drug delivery, drug release from the nanoplatforms cannot always be properly triggered to take place with the desired selectivity [27]. Moreover, homogeneous distribution and effective internalization of the drugs by the whole population of targeted cancerous cells are not always achievable [27]. Nevertheless, it would be possible to achieve sustained drug release over a prolonged period of time by modulating the porosity, surface charge, and biodegradability of the nanoplatforms .
The delivery of the nanoplatform to the target tissue can be achieved primarily in two ways—passive and active targeting (Fig. 1) [28]. Passive targeting takes advantage of the permeability of tumor tissue [28]. Due to rapid vascularization to serve fast-growing cancerous tissues, the capillary endothelium in cancerous tissue is more disorderly and thus more permeable toward nanoplatforms than the capillary endothelium in normal tissues. If the drug-loaded nanoplatform can stay in blood circulation for a reasonably long time, there will be enrichment of nanoplatforms into the tumor tissues. Furthermore, since the lymphatic system is not developed in tumor tissue, extravasated nanoparticles tend to stay inside the interstitial space in tumor tissues. This overall phenomenon of accumulation of nanoplatforms in tumor tissues is known as the enhanced permeability and retention (EPR) effect [28].
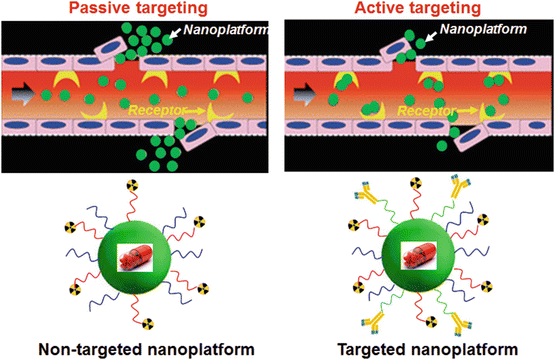
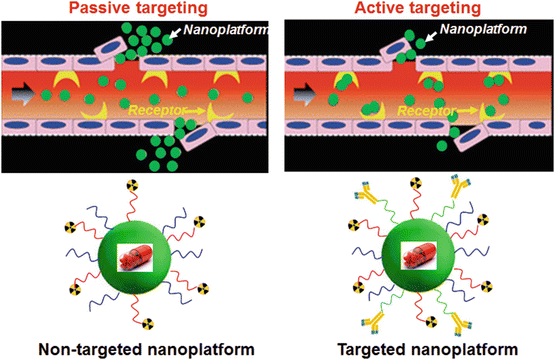
Fig. 1
Schematic depiction of EPR-mediated passive and active targeting using theranostic nanoplatforms. Nanoparticles can passively target tumors through preferential passage through larger interendothelial junctions compared to those of healthy tissues. Nanoparticles can also be conjugated with suitable targeting agents, such as antibodies that are specific to proteins (receptors) more highly expressed in tumors than healthy tissue, to actively target tumors. Adapted with permission from Ref. [28]
The EPR phenomenon is dependent on several factors such as particle size, particle surface charge and hydrophobicity, immunogenicity, tumor characteristics, etc., which results in many challenges in the optimization of passive targeting [28]. Further, specificity toward the tumor would be low in passive targeting and therefore therapeutic concentrations of the chemotherapeutic drug can be suboptimal at the tumor site resulting in poor therapeutic efficacy [11]. To overcome these limitations, active targeting would be a more viable approach that can be achieved by conjugating the functionalized nanoplatform to a suitable targeting moiety such as aptamers, peptides, or proteins, thereby allowing preferential accumulation of the drug in the tumor tissue [28]. The best results would be expected by combining the effects of both passive and active targeting to achieve maximal therapeutic efficacy [11].
A major hurdle in using nanoplatforms for image-guided drug delivery is their tendency to get trapped and cleared from the circulation by the reticuloendothelial system (RES) [27]. Additionally, nanoparticles can interact with plasma proteins effectively altering their surface properties [27]. The introduction of biocompatible hydrophilic polymer chains, such as polyethylene glycol (PEG) , creates a hydrated brush-like coating on the nanoparticle surface that enhances nanoparticle solubility, prolongs blood circulation times, and delays RES clearance [29, 30]. It has been demonstrated that PEG-coated nanoplatforms have circulation times several orders of magnitude longer than uncoated nanoplatforms [29, 30]. Various nanoplatforms have been radiolabeled with different positron emitting radioisotopes for PET image-guided drug delivery, most of which are summarized in Table 2 and discussed in the following text.
Table 2
Representative examples of theranostic nanoplatforms utilized for PET image-guided drug delivery
Drug carrier | Targeting ligand | Target | Therapeutic agent | PET isotope | Disease model | Tumor uptake | References |
---|---|---|---|---|---|---|---|
Liposome | None (passive targeting) | None (passive targeting) | Model hydrophilic drug | 18F and 64Cu | Met-1 tumors | (Not reported) | [33] |
Micelles | cRGD peptide | Integrin α v β 3 | Doxorubicin | 64Cu | Human glioblastoma | ~7 % ID/g | [37] |
Melanin nanoparticles | None (passive targeting) | None (passive targeting) | Sorafenib | 64Cu | Hepatocellular carcinoma | ~5.5 % ID/g | [26] |
Gold nanorods | cRGD peptide | Integrin α v β 3 | Doxorubicin | 64Cu | Human glioblastoma | ~6 % ID/g | [44] |
Mesoporous silica nanoparticles | TRC105 antibody | CD105 | Doxorubicin | 64Cu | Murine breast cancer | ~6 % ID/g | [49] |
3.1 Liposomes for PET Image-Guided Drug Delivery
Liposomes are good candidates as drug carriers and have been widely investigated in drug delivery systems [31, 32]. Basically, liposomes are self-assembled vesicles composed of a lipid bilayer, which forms a closed shell surrounding an internal aqueous phase. The major advantages of liposomal carriers for drug delivery are that they are biodegradable and nontoxic [31, 32]. Moreover, size, charge, and surface functionalization of liposomes are easily controllable and such systems are suitable for carrying both hydrophobic and hydrophilic drug molecules [31, 32]. Owing to these favorable characteristics, liposomes were the first nanoplatforms to make the transition from conceptual stage to clinical application, and are now an established technology platform with considerable clinical acceptance [7, 31, 32].
Paoli et al. reported the synthesis of liposomal formulations with particle size in the range 80–113 nm [33]. The liposomes were preconjugated with suitable fluorophores (calcein or AF-750) and radiolabeled with 18F or 64Cu for dual-modality PET/optical imaging. A model hydrophilic drug was encapsulated in the liposomal system and administered in mice bearing bilateral Met-1 tumors, to assess the relative stability and circulation kinetics of the drug-loaded liposomes, while maintaining temperature sensitivity. Using in vivo PET imaging and ex vivo fluorescent imaging of tumors, the authors could demonstrate that the accumulation of the drug was increased by up to 177-fold by liposomal encapsulation.
In a recent study, Lee et al. reported the synthesis of a chelator compound, 4-DEAP-ATSC, which serves as the 64Cu loading and entrapment agent in liposomal formulations [34]. The authors demonstrated that the 64Cu-DEAP-ATSC complex could be loaded into PEGylated liposomal doxorubicin (PLD) and HER2-targeted PLD (MM-302) with >90 % efficiency and that 64Cu-loaded liposomal formulations were stable in human plasma up to 24 h. In vivo PET imaging studies in BT474-M3 (HER2-overexpressing breast carcinoma) xenografts after administration of 64Cu-MM-302 showed heterogeneous distribution within tumors. The biodistribution profiles were quantitatively consistent with tissue-based analysis, and radioactivity uptake in the tumor (4.8 ± 0.7 %ID/g at 24 h postinjection) correlated with liposomal drug deposition. The promising results obtained in this study suggest that clinical translation of this strategy might aid in the identification of cancer patients who are most suited for undergoing liposomal therapy.
3.2 Micelles for PET Image-Guided Drug Delivery
Micelles are colloidal particles with a size usually within a range of 5–100 nm, and are currently under investigation for targeted delivery of hydrophobic anticancer drugs [35]. When tagged with suitable positron emitting radioisotopes, these systems can be used for image-guided drug delivery. Among the various micellar structures, the polymeric micelles are the most extensively used for drug delivery applications [35, 36]. The polymeric micelles generally consist of a unique core—shell structure. The inner core is the hydrophobic part of the block copolymer, which encapsulates the hydrophobic drug. The outer shell or corona of the hydrophilic block of the copolymer is often composed of PEG, and it protects the drug from the aqueous environment and also imparts particle stability and excellent dispersibility in an aqueous solution. Because of these characteristics, polymeric micelles have several advantages as drug carriers such as enhancing the aqueous solubility of hydrophobic drugs, prolonging the circulation time of the drug in the blood, improving the in vivo stability of the drug, providing both passive and active tumor targeting abilities, and reducing nonspecific uptake by the RES [35, 36].
In the first use of micellar systems for PET image-guided drug delivery in tumor-bearing mice, Xiao et al. reported the synthesis of multifunctional unimolecular micelles made of a hyperbranched amphiphilic block copolymer [37]. The hyperbranched block copolymer of the micellar system was conjugated with cyclo(Arg-Gly-Asp-D-Phe-Cys) peptides (cRGD , for integrins α v β 3 targeting) and macrocyclic chelators [1,4,7-triazacyclononane-N,N′,N″-triacetic acid (NOTA)], for 64Cu-labeling. An anticancer drug , doxorubicin (DOX ), was also covalently conjugated onto the hydrophobic segments of the amphiphilic block copolymer arm to enable targeted drug delivery and pH-controlled drug release. In vitro studies showed that cRGD-conjugated unimolecular micelles exhibited a much higher cellular uptake in human glioblastoma (U87MG) cells due to integrin α v β 3-mediated endocytosis compared to nontargeted unimolecular micelles, thereby leading to a significantly higher cytotoxicity. In vivo PET imaging and biodistribution studies in U87MG (human glioblastoma) xenografts revealed that targeted unimolecular micelles (conjugated with cRGD peptide) exhibited a much higher level of tumor accumulation (~5 %ID/g) than nontargeted unimolecular micelles (~2.5 % ID/g) at 4 h postinjection (Fig. 2a). Administration of a blocking dose of cRGD peptide (10 mg/kg of mouse body weight) followed by administration of radiolabeled unimolecular micelle conjugated with cRGD peptide reduced the tumor uptake significantly (~2 % ID/g), which confirmed integrin α v β 3 specificity of the targeted micelle in vivo (Fig. 2a). These results were further confirmed by ex vivo optical imaging using the fluorescence signal of DOX (Fig. 2b).
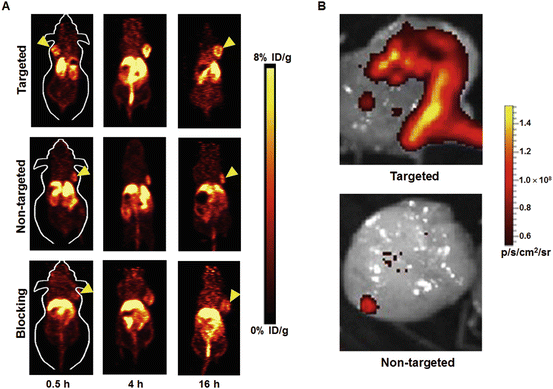
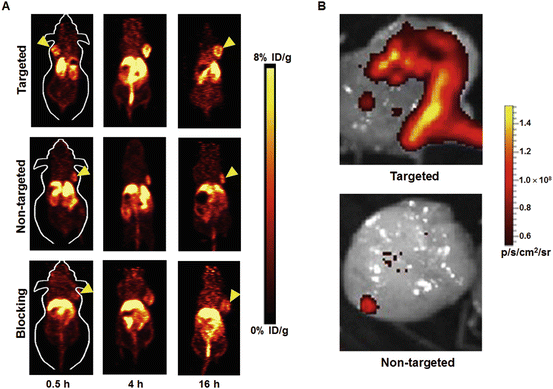
Fig. 2
PET image-guided drug delivery using unimolecular micelle. (a) PET imaging of U87MG tumor-bearing mice at different time points postinjection of 64Cu-labeled unimolecular micelle loaded with DOX (nontargeted), 64Cu-labeled unimolecular micelle conjugated to cRGD and loaded with DOX (targeted), and 64Cu-labeled unimolecular micelle conjugated to cRGD and loaded with DOX with a blocking dose of cRGD (blocking). (b) Ex vivo fluorescence imaging of U87MG tumor, with the excitation and emission set for detecting DOX fluorescence, harvested from mice injected with targeted and nontargeted unimolecular micelles. Adapted with permission from Ref. [37]
The same group of authors further extended the work by conjugation of anti-CD105 monoclonal antibody (TRC105) with the unimolecular micelles (instead of cRGD peptide as done in the previous study) [38]. TRC105-conjugated unimolecular micelles showed a higher CD105-associated cellular uptake in human umbilical vein endothelial cells (HUVEC) compared with nontargeted unimolecular micelles. Similar to the previous study, in vivo PET imaging and biodistribution studies in 4T1 murine breast tumor-bearing mice showed that a tumor accumulation of ~6 % ID/g of targeted micelles (i.e., conjugated with TRC105) was higher than that of nontargeted micelles (~3 % ID/g) at 5 h postinjection. In a recent study, the same group of authors reported the development of a new type of unimolecular micelle formed by brush-shaped amphiphilic block copolymers [39]. As in the previous study, the unimolecular micelle was conjugated with TRC105, loaded with DOX and radiolabeled with 64Cu for PET image-guided drug delivery in 4T1 tumor-bearing mice and similar results were obtained. The encouraging results obtained in all these studies clearly indicate that unimolecular micelles are promising form of nanomedicine for targeted cancer theranostics .
3.3 Endogenous Nanosystems for PET Image-guided Drug Delivery
Over the last few years, there is growing interest toward the use of endogenous organic nanostructures, such as ferritins, melanin, etc. as drug delivery platforms due to their native biocompatibility and biodegradability [26, 40, 41]. In this direction, it is desirable to develop endogenous systems that intrinsically possess both contrast and drug delivery properties. Recently, Zhang et al. reported the synthesis of melanin nanoparticles as an efficient endogenous system for multimodality image-guided drug delivery [26]. Melanin is a biopolymer with good biocompatibility and biodegradability, intrinsic photoacoustic properties, and binding ability to various types of chemotherapeutic drugs [41]. The synthesized nanoparticles were PEGylated, loaded with an anticancer drug (Sorafenib ), radiolabeled with a 64Cu adopting chelator-free approach, and then used for dual modality PET and photoacoustic image-guided drug delivery. In vivo PET imaging and biodistribution studies after intravenous administration of the nanoplatforms in hepatocellular carcinoma (HepG2) tumor-bearing mice revealed rapid tumor uptake by passive targeting (5.5 ± 0.3 % ID/g at 4 h postinjection) with a good tumor to background contrast. The results of PET imaging were further corroborated by photoacoustic imaging. Furthermore, the authors could successfully demonstrate the antitumor effects of drug-loaded melanin nanoparticles by studying the inhibition of tumor growth in vivo. The promising results obtained in this study prove that melanin nanoparticles are an efficient biosystem for multimodality image-guided drug delivery and hold potential for clinical translation in the foreseeable future.
3.4 Metallic Nanoparticles for PET Image-Guided Drug Delivery
Among the various metallic nanoparticles reported to date, gold nanostructures possess unique characteristics that enable their use as contrast agents, therapeutic entities, and frameworks to attach functional molecules, therapeutic cargo, or targeting ligands [42, 43]. Owing to their ease of synthesis, easy surface functionalization, and nontoxicity, gold nanostructures have emerged as powerful nanoplatforms for cancer theranostics [42]. The development of a multifunctional gold nanorod-based nanoplatform for PET image-guided drug delivery was reported by Xiao et al. [44]. The bare gold nanorods had a length and diameter of approximately 45 and 10 nm, respectively. The gold nanorods were PEGylated and an anticancer drug (DOX ) and tumor targeting agent (cRGD ) were conjugated to it. A chelator, NOTA, was attached onto the distal ends of the PEG arms for 64Cu labeling. Based on flow cytometry analysis, cRGD-conjugated gold nanorods loaded with DOX exhibited a higher uptake and cytotoxicity in U87MG cells compared to nontargeted gold nanorods in vitro. However, in vivo PET imaging and biodistribution studies showed that targeted and nontargeted gold nanorods had a similar distribution pattern, in particular in respect to tumor uptake (~5 % ID/g). Despite this limitation, this study demonstrated the potential of gold nanorods as an efficient nanoplatform for cancer theranostics .
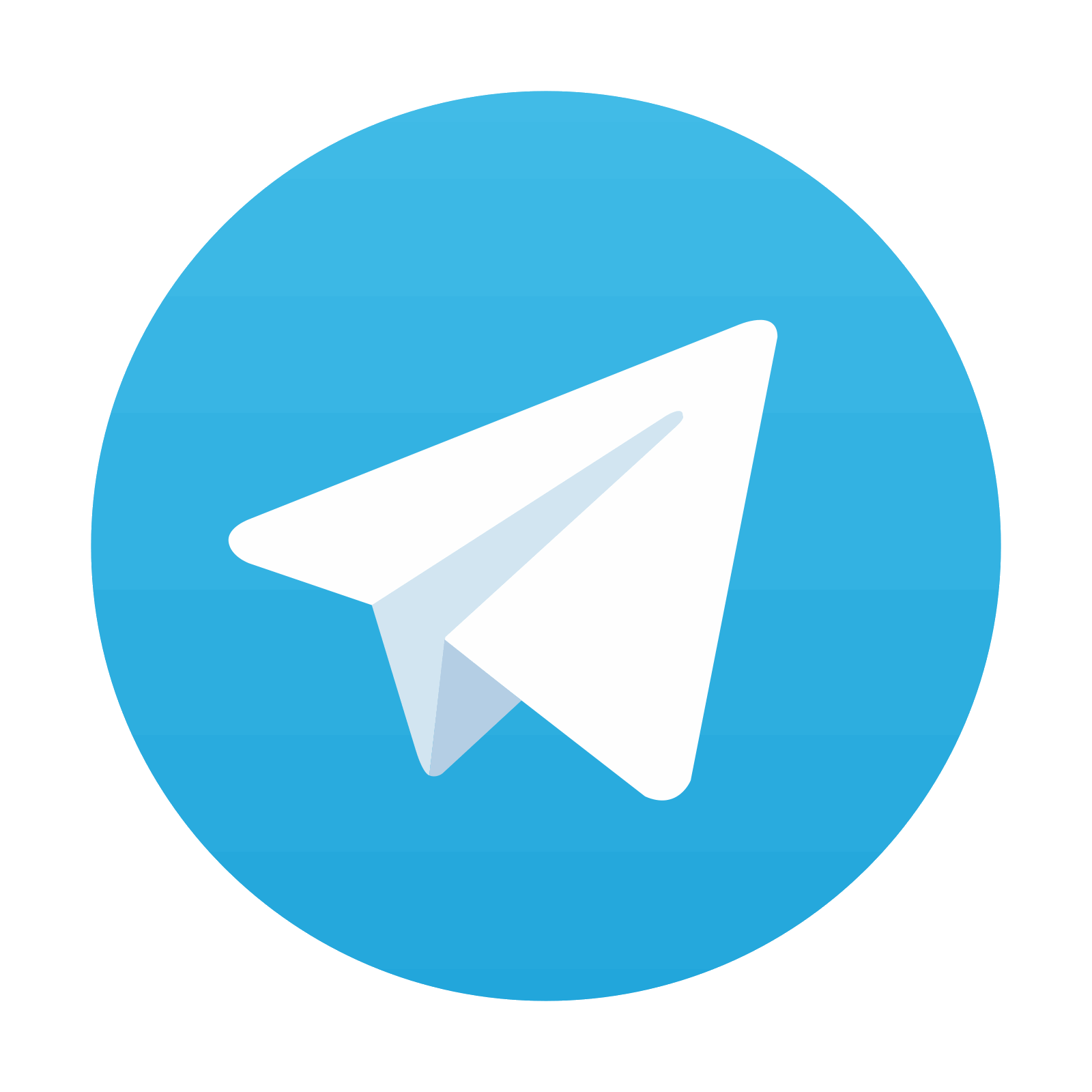
Stay updated, free articles. Join our Telegram channel
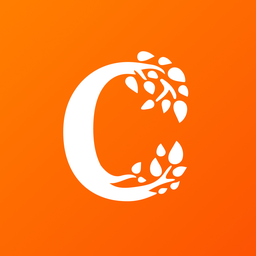
Full access? Get Clinical Tree
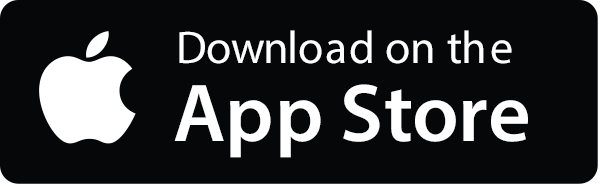
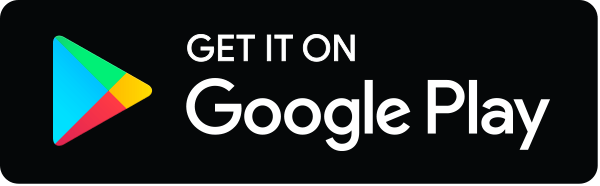