Fig. 7.1
Ionizing energy transmission associated with radiation particles (Adapted from “A Small Dose of Toxicology”, with permission)
Beta particles, in contrast, are high-energy, high-speed positrons or electrons that emit ionizing radiation in the form of beta rays. The production of beta particles is termed beta decay. There are two forms of beta decay, β− and β+, which respectively give rise to the electron and the positron. Of the three common types of radiation given off by radioactive materials, alpha (Fig. 7.1), beta, and gamma, beta has the medium penetrating power and the medium ionizing power. Although the beta particles given off by different radioactive materials vary in energy, most beta particles can be stopped by a few millimeters of metal such as aluminum or lead. Since it is composed of charged particles, beta radiation is more strongly ionizing than gamma radiation. Beta radiation is used to treat some malignancies, and beta decay is a source of positrons for PET (positron emission tomography) scans where a radiolabeled sugar (fludeoxyglucose) emits positrons that are converted to pairs of gamma rays to localize malignancies since they are typically more metabolically active than other surrounding tissues.
Gamma radiation is a high-frequency, high-energy radiation typically produced by the decay of atomic nuclei in high-energy states such as radium. Unlike alpha and beta particles, gamma rays represent a form of radiation rather than a source of radiation. Gamma rays are distinguished by x-rays by their source of origin; gamma rays are emitted by atomic nuclei, whereas x-rays are emitted by electrons [3]. Protection from gamma rays requires large amounts of mass in contrast to beta and alpha particles. Gamma radiation is used in imaging technologies such as PET scans. Other uses include technetium 99-m that emits gamma rays in the same energy range as diagnostic x-rays. During a technetium 99-m scan, a gamma camera can be used to form an image of the radioisotope’s distribution by detecting the gamma radiation emitted.
X-rays are a form of electromagnetic radiation with a wavelength range of 0.01–10 nm with associated energies in the range 100 eV–100 keV. The wavelengths are shorter than those of ultraviolet radiation and typically longer than gamma radiation. X-rays are useful in imaging technology because they can penetrate tissue without significant absorption or scattering. X-ray interaction with matter for the purposes of imaging modalities occurs through photoelectric absorption.
Exposures to ionizing radiation include air travel; this increases our exposure to cosmic and solar radiation that is normally blocked by the atmosphere. Radiation intensity is greater across the poles and at higher altitudes, thus individual exposure varies depending on the route of travel. Storms on the sun can produce solar flares that can release larger amounts of radiation than normal. For the occasional traveler, this radiation exposure is well below recommended limits established by regulatory authorities. However, frequent fliers and airline workers can be exposed to levels of radiation that exceed established guidelines.
Sources of ionizing radiation or exposed populations:
Medical x-ray devices (patients, health-care employees)
Radioactive material producing alpha, beta, and gamma radiation (laboratory workers, health-care employees, patients)
Cosmic rays from the sun and space (air travel)
Radiation Units
The units used to describe exposure and dose of ionizing radiation to living material are confusing, at best. First, the units have changed to an international system, SI (Systeme Internationale). The subsequent description will use the SI system. Different methods exist to measure radiation. The radiation dose that the patient experiences can be measured directly. So, while the fundamental descriptive unit of ionizing radiation is the amount energy, expressed in coulombs or joules per kilogram of air, and is the unit of exposure in air, the absorbed dose is the amount of energy absorbed by a specific material such as the human body and is described as the gray (Gy), previously the rad (radiation absorbed dose). The gray is used to assess absorbed dose in any material. One gray delivers 1 J of energy per kilogram of matter. However, the energy transfer of the different particles and gamma rays is different. A weighting factor is used to allow comparison between these different energy transfers. Further, tissues and organs have different sensitivities to radiation. As a consequence, equivalent and effective dose concepts were developed.
The unit for the equivalent dose is the sievert (Sv). The Sv is used to estimate the stochastic (see below) biologic effect of ionizing radiation on tissue and has an equivalent, effective and committed dose weighted averaging for each biologic tissue. For example, while 1 Sv = 1 J/kg = 1 Gy, the absorbed dose of 1 mGy of alpha radiation would be equal to 20 mSv because of the weighting factors of alpha radiation. A further refinement is possible that applies a weighting factor to each type of tissue. Recommended limits on radiation exposure are expressed in sieverts. Radiation imaging exposure units include milliampere-seconds (MAS).
Air kerma rate is also used to as a radiation unit. Kerma (kinetic energy released in matter) represents the kinetic energy absorbed per unit mass of a small amount of air when it is irradiated. It is associated only with indirectly ionizing radiation and is used as a replacement quantity for absorbed dose when the absorbed dose is difficult to calculate such as in fluoroscopy. Air kerma rate is the rate calculated using (u/p) value for air and is measured in Gy per unit time (Gy/h).
Several derivations on this unit exist including air kerma–area product (Pka) and air kerma at the reference point (Ka,r) [4]. Cumulative dose (CD) represents the air kerma accumulated at a specific point in space relative to the interventional reference point (typically the fluoroscope gantry). This is also referred to as cumulative air kerma. Other units include dose–area product (DAP). This measurement represents the integral of air kerma across the entire x-ray beam emission and serves as a surrogate measurement for the entire amount of energy delivered. DAP is measured in Gy · cm2.
Health Effects: Ionizing Radiation
Ionizing radiation is more harmful that nonionizing radiation because it has enough energy to remove an electron from an atom and thus directly damage biological material. The energy is enough to damage DNA, which can result in cell death or induce cellular neoplastic change (cancer). The study of ionizing radiation is a large area of classical toxicology, which has produced a tremendous understanding of the dose–response relationship of exposure. The primary effect of ionizing radiation resides in its effect on DNA. It can also affect the developing fetus of mothers exposed during pregnancy. Radiation exposure has a direct dose–response relationship.
Our knowledge of the effects of radiation developed gradually from experience over the last century. Early in the century, researchers such as Marie Curie died of cancer possibly related to her radiation exposure. Occupational exposure has also informed our understanding of radiation exposure risks. Young women employed to paint radium on watch dials died from bone cancer in the 1920s and 1930s [5, 6]. During this time, radium was promoted as a cure of many maladies and even recognized by the American Medical Association as a therapeutic option.
A great deal was learned from the atomic bomb survivors at Nagasaki and Hiroshima. The US military dropped the first atomic bomb on Hiroshima, Japan, on August 6, 1945, and a second on Nagasaki, Japan, 3 days later. The bombs used two different types of radioactive material, 235U in the first bomb and 239PU in the second. It is estimated that 64,000 people died from the initial blasts and radiation exposure. Approximately 100,000 survivors were enrolled in follow-up studies, which confirmed an increased incidence of cancer. Ionizing radiation was also used to treat disease. From 1905 to 1960, ionizing radiation was used to treat ringworm in children and ankylosing spondylitis as well [7]. Experience with the use and misuse of ionizing radiation has demonstrated that the greater the dose, the greater the likelihood of developing cancer and that latency periods need to be measured in decades (from 10 to 60 years).
Medical Imaging
Medical imaging has become so commonplace in the United States and other resource-rich countries that the medical standard of care necessitates its use. The last two decades have seen the advancement and popularization of new imaging modalities such as magnetic resonance imaging (MRI), positron emission technology (PET) in addition to the standard use of fluoroscopy, and ultrasound technologies. It is a rare patient who has not received any imaging studies. With the widespread use of prenatal ultrasonography, most young people have experienced an imaging study even prior to birth. While ultrasound-imaging technology does not use ionizing radiation, some health concerns exist around its use. The Food and Drug Administration (FDA) has set limits on exposure at 4 T for infants less than 1 month old and 8 T for adults [8].
Nonionizing Modalities
Some modalities like ultrasonography and MRI are not associated with ionizing radiation and so are considered to have low to nonhazardous health risks to humans. The high-magnetic fields used in clinical MRI range up to 3 T. Ultrasonography employs high-frequency sound waves for visualization. The power levels used for imaging are currently believed to be below the threshold to cause short-term or long-term tissue damage.
The long-term effects due to ultrasound exposure at diagnostic intensity are still unknown, but ultrasonography as a diagnostic modality has been used in increasing frequency over the last half century [8]. The ALARA (as low as reasonably achievable) principle has been employed in this field of radiology—to minimize scanning time and power settings as low as possible while still achieving imaging goals. Nonmedical uses are discouraged under this principle as well.
Nonionizing imaging modalities are the imaging modalities of choice for children because of the recognized risks associated with ionizing radiation and cancer mortality [9]. However, ionizing radiation modalities provide diagnostic ease and clarity that cannot be reproduced by nonionizing modalities and where the risk–benefit ratio clearly rests on the side of using the study [10].
Ionizing Modalities
Radiology modalities that involve the use of ionizing radiation include diagnostic fluoroscopy, nuclear medicine imaging, and computerized tomography (CT) [11]. While the use of all these imaging modalities has increased over time, the use of CT in pediatrics has increased particularly rapidly largely because of the advent of helical CT which allows for increased accuracy in imaging over a shorter period of time [9]. This allows one to avoid the need for sedation to produce a useful study. The increase in exposure from ionizing radiation imaging modalities has increased 600 % from 1980 to 2006 (0.54–3 mSv) with medical radiation now accounting for half of the total radiation exposure in the United States. Repeated postnatal exposure of children to ionizing radiation to evaluate scoliosis is associated with increased rates of breast cancer later in life. This has raised concern for increased risk for other malignancies as well [12, 13].
Concern for increased lifetime risk for malignancies secondary to radiation exposure from these modalities was brought to attention in the late 1990s. Cancer risk estimations for children exposed to a CT (using adult radiation exposure doses) were calculated by Brenner who found a 0.18 % (abdominal) and 0.07 % (head) increased lifetime risk for cancer. These percentages were an order of magnitude higher in children than in adults based on the increased lifetime risk-dose exposure. These estimates were derived from cancer risk calculations and mortality data from atomic bomb survivors in Japan. That data demonstrated increased cancer mortality data with doses greater than 100 mSv with decreasing risk for lower radiation exposures [14–18].
Risk Assessment
Radiation risk can be considered in the following categories:
1.
Stochastic risk to the individual
2.
Stochastic risk to society
3.
Deterministic risk to the individual
4.
Pregnancy exposure-related risks
Deterministic risks represent radiation-induced tissue damage that manifests itself within days to weeks after exposure. This includes radiodermatitis and radiation-induced skin ulceration. Interventional fluoroscopic procedures represent the most common mechanism for this type of exposure. In particular, complex interventional procedures with prolonged fluoroscopy times increase deterministic risks associated with ionizing radiation exposure. Deterministic effects of tissues such as the skin, lenses of the eyes, and hair follicles are a by-product of damage to supporting tissues and sterilization of stem cells. Tissue damage occurs when the ability of the affected organ to repair itself by cellular division is overwhelmed by the cell loss due to radiation damage. Lethal levels of radiation are used to intentionally kill cancer cells. Consequently, the extent and timing of tissue damage is related to cell proliferation kinetics of the irradiated organs [19]. Deterministic risks are uncommon in general and even more uncommon in pediatrics [20, 21]. Deterministic effects occur at high doses over short exposure times and are usually seen at doses over 0.1 Gy or high-dose rates (0.1 Gy/h).
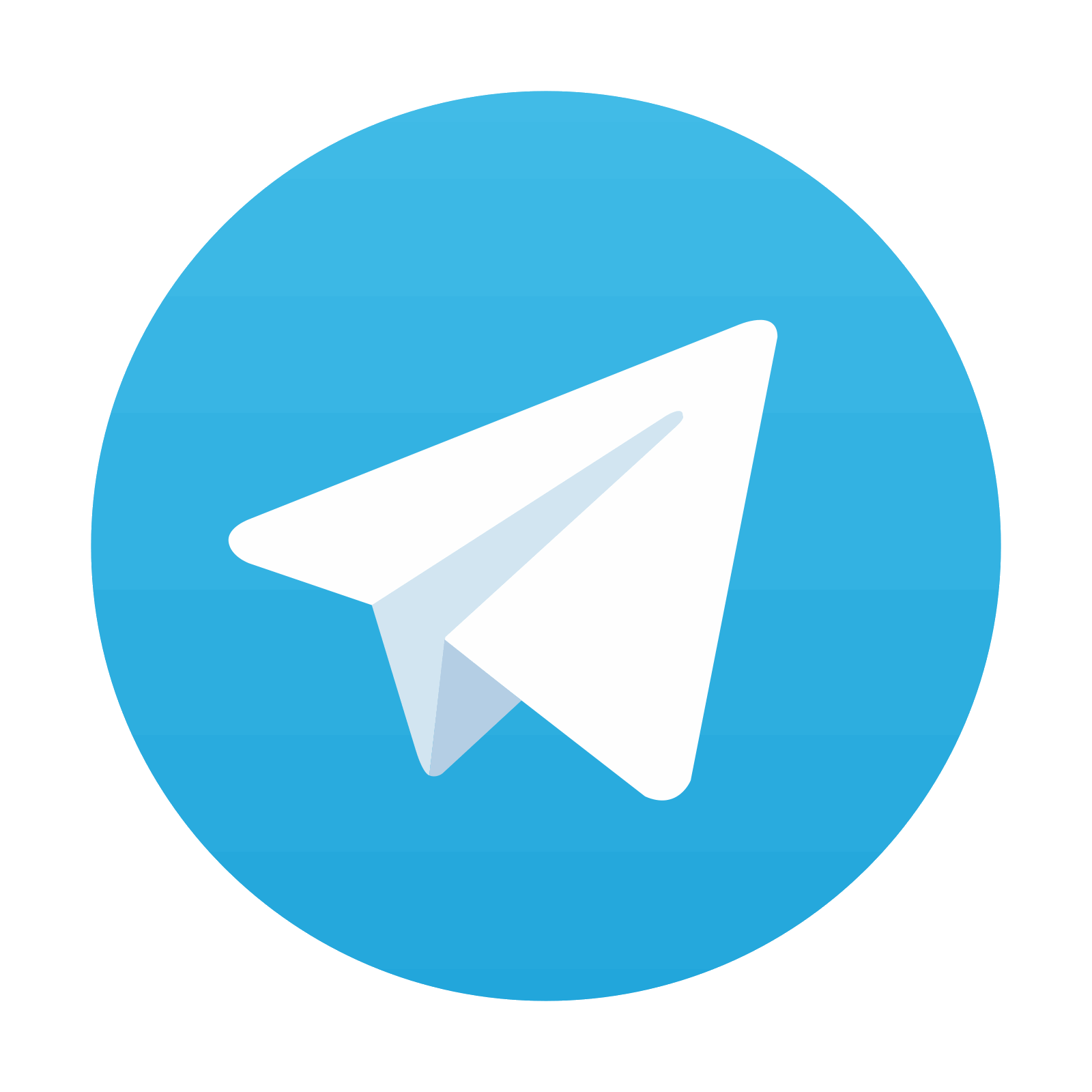
Stay updated, free articles. Join our Telegram channel
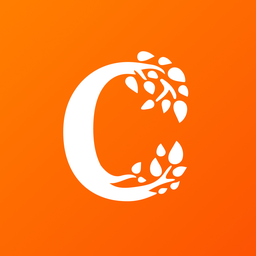
Full access? Get Clinical Tree
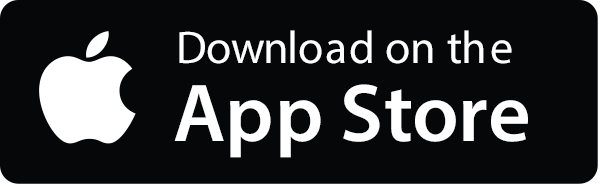
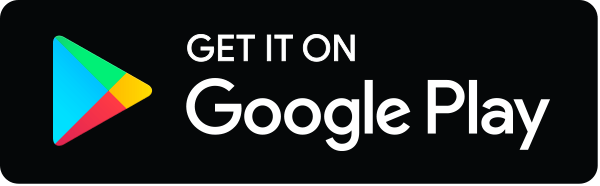