Fig. 1
Serial whole-body scans after intrathecal injection of 0.5 mCi of In-111 DTPA via an Ommaya reservoir (a), or via lumbar puncture (b). Patency of CSF is demonstrated in both cases and subsequent intrathecal chemotherapy was uneventful. This scheme provides an opportunity to evaluate CSF kinetics and dosimetry in one setting
One example of intracavitary radionuclide therapy is the application of Y-90 labeled DOTA-TOC to treat post-surgical cavities in patients with gliomas. Under the surrogate guidance of In-111 labeled octreotide, serial images demonstrated prolonged retention of In-111 octreotide in the tumor bed (Fig. 2, courtesy of Drs. Heute and Virgolini, Medical University of Innisbrook, Austria). More interesting is the visualization of CSF compartments and subsequent systemic return from both interstitial and intrathecal routes. Good responses to Y-90 DOTA-TOC were reported in this preliminary study (Heute et al. 2010), indicating justification of interstitial/intracavitary treatment of brain tumors using unsealed soluble radiopharmaceuticals. Other intracavitary radionuclide therapies inside the pleural, pericardial, peritoneal compartments or postsurgical tumor bed also require radiographic and/or scintigraphic confirmation of adequate distribution of injectate, typically using Tc-99m MAA or sulfur colloids. Intracavitary radiopharmaceuticals may have limited diffusion outwards to ablate surrounding micrometastases.
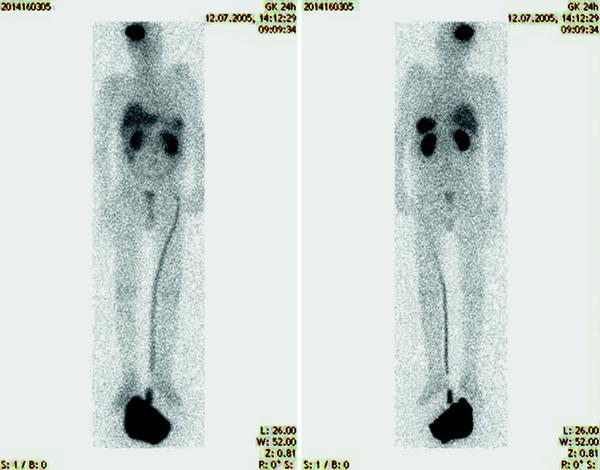
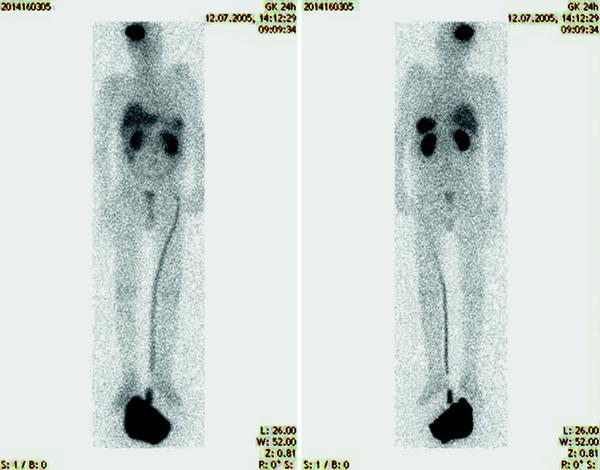
Fig. 2
Whole-body scan 24 h after a patient received 0.5 mCi of In-111 octreotide via an intracavitary manner to evaluate distribution of In-111 octreotide in a patient with demonstrated somatostatin receptors in the resected glioma. There is retention of tracer in the cavitary although there is also significant return of tracer to systemic circulation as demonstrated by tracers in the liver, spleen, kidneys, and urinary collection (courtesy of Dr. Heute)
Interstitial injection of particulate radiopharmaceuticals into tumor bed is usually limited by loculation around the injection site and concentration of radiation in very confined areas. It may necessitate multiple injections in different regions of the tumor. For instance, after Y-90 Y(OH)3 particles were injected in human superficial tumors in multiple locations within a tumor, there is significant local control (Asakura 1964). Small portions (<5 %) of particles of smaller sizes, e.g., below 1 μm may be drained via the lymphatic system but obliteration of the sentinel lymph node is not a limiting factor for their therapeutical use. Soluble radiopharmaceuticals such as Y-90 DOTATOC (Heute et al. 2010) may diffuse from the injection site to neighboring tissues before returning to the systemic circulation via the capillary bed and still be able to ablate more distant tumors from the injection site or cavity. The interstitial use of soluble radiopharmaceuticals with tumor affinity may enhance the retention of radionuclide, resulting in more delivery of the payload. This latter approach remains to be further studied.
1.3 Locoregional Versus Systemic Radiobiology: Efficacy and Toxicity
The overall goal of cancer treatment is to eradicate primary and metastatic tumors and to prevent recurrence. Locoregional radionuclide therapy can provide effective local control of primary or metastatic tumors, depending on the site of administration. Local diffusion of radionuclide may be able to reach micrometastases around the injection site. Distant metastases, either bulky or microscopic usually cannot be adequately treated because of the relatively small amounts of systemic return of radionuclide. On the other hand, systemic therapy may deliver radionuclide to both primary and metastatic sites to a similar extent because the delivery is mainly dependent on vasculature and cancer phenotypes. Presumably, micrometastases are also exposed to radionuclide to similar extents although the efficacy of eradicating micrometastases is doubtful and needs to be correlated with microdosimetry.
The usefulness of radionuclide therapy is limited by radiation absorbed doses to critical organs. Because of the vast pharmacokinetic advantage of locoregional radionuclide therapy resulting in wide therapeutic windows, high radiation absorbed doses (e.g., >100 Gy) can be delivered to the tumor bed, as long as the diffusion to surrounding tissue is slow and the resultant toxicity to surrounding tissue is low. The higher radiation absorbed doses will result in more complete ablation of tumors leading to apoptosis and/or concomitant necrosis. When tumor cells are exposed to lethal doses of radiation in the range (100–200 Gy) of locoregional radionuclide therapy, they become metabolically inactive with no immediate structural damage to cells or stoma. Since protein inactivation occurs at higher absorbed doses (~100,000 Gy), tumor cells and surrounding tissues will retain structural integrity initially. However, tumor defense such as autocrine formation to resist host immunologic surveillance will be suppressed. Tumor-specific antigens will be presented to host immune systems including macrophage recognition and amplification mechanisms leading to eventual tumor destruction by macrophages or cytotoxic mechanism. Along this rationale, nonradioactive locoregional tumor therapeutic experiments such as photodynamic therapy (Garg 2010; Korbelik 2010) and radiofrequency ablation have reported increased specific immune responses toward tumors (Saji et al. 2006). On the other hand, locoregional external beam irradiation after mastectomy is correlated with decreased lymphocytes in breast cancer patients (Koukourakis et al. 2009). Locoregional radionuclide therapy may have an important and perhaps optimal role because there are no immediate physical damages to the tumor or surrounding tissues, allowing a window of opportunity of exposure and recognition of tumor-specific antigens without interference by locoregional immune resistance. The ideal end result will be eradication of tumor cells with less nonspecific inflammation of surrounding tissues, and the triggering memory mechanisms for host defense upon future recurrence.
Competent immune mechanisms may be less operative with conventional chemotherapy because often the immune mechanisms are also suppressed. In theory, this benefit could be shared by systemic radionuclide therapy and external beam radiotherapy including proton beam or stereotactic radiotherapy. However, narrower therapeutic windows of these latter therapeutic modalities may limit radiation absorbed doses to the tumor bed to achieve metabolic ablation and to overcome locoregional immune resistance.
1.4 Radiopharmaceuticals: Radionuclide Ranges and Residence Time
Radionuclide’s emitting alpha (Bdeir et al. 2010; Groves et al. 1995), beta (Heute et al. 2010; Lee et al. 2002) and gamma rays (Ljunggren et al. 2004) have been used in locoregional therapy. Each radionuclide has the characteristic range and physical half-life and each radiopharmaceutical has unique biologic residence time in each organ or tissue. While the range depends on the energies of all the emissions, the residence time is governed by biokinetics and may be measured by scintigraphy or emission tomography. Therefore, the choice of radiopharmaceuticals for locoregional application depends on locoregional pharmacokinetics which also determines dose rate and irradiation duration.
The choice of radionuclide depends on ranges of target to be ablated and important normal organs or critical organs to avoid. Monte Carlo simulation of spheres filled with radionuclide allows estimation of radiation absorbed doses inside the volume of the injectate (Fig. 3). These factors, together with total administered radioactivity and residence time estimate from centigrams directly determine the radiation absorbed doses in the target and the surrounding tissues and critical organs. In theory, the ideal radiopharmaceuticals will deliver high radiation absorbed dose to the target tumor and low doses to the critical organs. For instance, radioiodide (I-131) ablation of thyroid cancer remnants delivers very large radiation dose (>100 Gy) to the target because the residence time in the remnant is usually long (5–8 days) while other organs such as bone marrow and kidneys are usually spared because the residence times are small in those organs (<1 h). In summary, radiation dosimetry to target tumor and critical organs as well as depth dosimetry to surrounding tissues are the prime considerations for choice of radiopharmaceuticals for locoregional therapy.
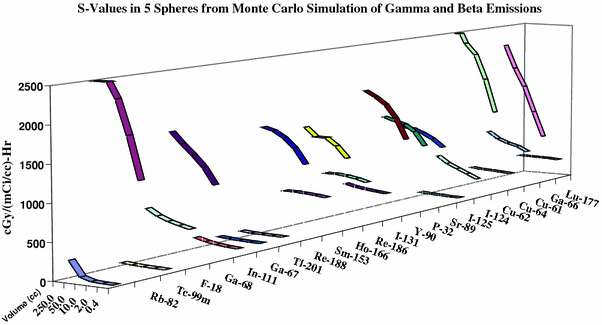
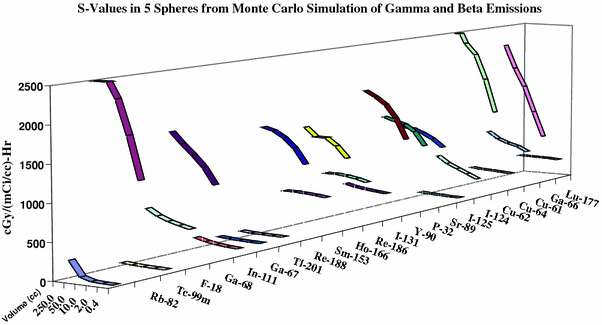
Fig. 3
Monte Carlo simulation of radiation absorbed doses for spheres of 5 volumes of 22 radionuclides. There is a predictable relationship between the volume of the sphere and the dosimetry allowing treatment planning
In practice, radiation absorbed doses can only be estimated by modeling as stated above because there are no reliable methods to measure them directly during radionuclide therapy. Certainly, accurate definition of target tissue shape and volume is also important in the estimation of radionuclide distribution in the target and for radiation dosimetry calculations. Therefore, scintigraphy, emission tomography, and hybrid imaging are necessary to obtain image-derived radiation dosimetry to verify the initial estimates and to correlate with treatment responses.
1.5 Radiation Dosimetry: Modeling and Limits
Accurate estimation of radiation absorbed doses to tumor, surrounding tissues, and critical organ is important by itself and in the design and modification of dosing regimes. Although a general-purpose FDA-approved radionuclide dosimetry software OLINDA (Stabin et al. 2005) is available for systemically administered radiopharmaceuticals, locoregional administration requires custom-design dosimetry schemes to estimate the target dose and the dose to the surrounding tissues. Monte Carlo simulations with anthropomorphic models are often applied but are rigidly limited to standardized patients and require lengthy computation sessions (Sparks et al. 2002). No post-processing is possible and therefore each modification of initial morphological assumptions or sets of regions of interests requires a repeated lengthy simulation session. In spite of these limitations, different models of intratumoral and intrathecal radionuclide therapy have been reported. A later development is the use of deterministic approach using a priori criteria to perform simulation (Vassiliev et al. 2008; Gifford et al. 2008). This deterministic approach allows prompt post-processing to modify initial estimation but is computation intense. Using this approach, radiation dosimetry for external beam radiotherapy can be estimated within 1–2 % variability. The accuracy of this approach in radionuclide therapy has not been well studied. Since image-based radionuclide therapy dosimetry is subject to larger variability at 14–25 % (Flower and McCready 1997), the use of dosimetry in radionuclide therapy should be guarded and at best used as a guidance tool. In fact, there is also doubt whether radiation dosimetry to the whole tumor is the whole answer to predict locoregional radionuclide therapy. For instance, small intratumoral activity of Tl-201(0.5 mCi) delivering 2–8 Gy was able to eradicate brain tumor implants in rats (Ljunggren et al. 2004). Other considerations such as accuracy in estimation of injectate volume, geometry of injectate, and microdosimetry to subcellular organelles or subcellular distribution of radionuclide need to be further studied for better correlation with therapeutic efficacies.
2 The Practice of Locoregional Radionuclide Cancer Therapy
2.1 Intrathecal Treatment of Meningeal Carcinomatosis
Meningeal carcinomatosis or meningeal metastases remains one of the most difficult management problems of lethal cancer complications. The incidence varies among different cancer types but may be as high as 10 % in melanoma patients and 25 % in leukemia. Successful eradication of tumor cells from the subarachnoid space is an effective means of controlling morbidity and mortality as in the case of intrathecal chemotherapy of acute leukemia in children. However, intrathecal chemotherapeutics are associated with large physical mass of therapeutics (many milligrams each time) and limited by slow diffusion, arachnoiditis, and other locoregional neurotoxicity in CSF compartments. Treatment using external beam radiation is generally limited to 30 Gy as palliative measures, because of the brain and spinal cord surrounding the arachnoid tumor deposits.
Intrathecal radionuclide may be able to deliver sufficient local radiation absorbed doses (e.g., over 50 Gy) to the arachnoids to ablate tumors with short-range radiations that do not require diffusion nor reach nervous tissues a few millimeter from the CSF interface. Chemical arachnoiditis may be avoided using minute amounts of carrier molecules or no-carrier-added radionuclide because of the generally small physical mass (<1 ng) required for the radionuclide itself. Considerations of intrathecal radionuclide therapy include high locoregional dosimetry to the arachnoid, low neurotoxicity, and low systemic toxicity. Therefore, design of intrathecal radionuclide therapy may be able to explore radionuclide therapeutics of known low systemic toxicities, as long as temporal and spatial profiles of their intrathecal pharmacokinetics are identified and validated.
Intrathecal introduction of radionuclide has been reported with different radionuclide including I-131 radioiodinated serum albumin (RISA), In-111 DTPA, Tc-99 m DTPA, I-131 sodium iodide, and Yb-192 DTPA for diagnostic purposes. Typically, radiopharmaceuticals are injected via lumbar puncture. Because of more comprehensive biodistribution to CSF space by intraventricular injections and because of availability of Ommaya reservoirs to perform repeated CSF aspiration, more intrathecal injections are done in this manner. Whether injection occurs in the intraventricular space or intralumbar space, a cisternogram (e.g., Fig. 1) using 0.5 mCi In-111 DTPA is recommended before the injection to assure CSF patency and to avoid loculation. Therapeutic trials using radionuclide with no tumor affinity such as 3 mCi P-32 chromic phosphates (Muriel et al. 1976; Tautz et al. 1977) or 1.5–5 mCi of Au-198 colloid (Brunhober 1981; Doge and Hliscs 1984) have been reported with efficacy but suffered from cauda equine syndrome. Tumor targeting I-131 labeled monoclonal antibodies (MoAb) (Moseley et al. 1990; Richardson et al. 1990; Benjamin et al. 1989; Kemshead et al. 1996; Kramer et al. 2000) and tumor basement matrix tenascin-targeting MoAb 81C6 up to 80 mCi with 60 mg protein content have been reported. Efficacies have been reported along with limiting hematotoxicity from the circulating radioiodinated antibodies and metabolites (Bigner et al. 1995). With locoregional sequestration of radionuclide in the CSF space, the question of whether tumor affinity of the radionuclide is necessary or whether it indeed confers significant advantages over adverse effects still remains to be studied. Inspection of CSF pharmacokinetic models and radiation dosimetry estimates has led to phase I trials using I-131 sodium iodide up to 120 mCi, injected via an intraventricular or an intralumbar approach. Preliminary findings of favorable safety and efficacy profiles have been reported in ASCO. This approach of using established systemic radiopharmaceuticals for locoregional radionuclide therapy will shorten the translation cycle from clinic to the bench and then back to the clinic. Another interesting parallel example is to explore theoretical models comparing intrathecal dosimetry of I-131 and Ga-67 (van Dieren et al. 1994). The results suggest favorable dosimetry for intrathecal use of Ga-67 because of the abundant Auger electrons. The establishment of theoretical dosimetry models followed by imaging for verification and derivation of residence times is indeed a feasible approach for the development of future intrathecal radionuclide therapy.
2.2 Pleural and Pericardial Effusion
Pleural effusion is a common complication of cancer from the lungs, breast, lymphoma, or other tumors affecting large volumes of the lungs. The majority of pleural fluid is produced by the parietal pleura covering the structure of the thoracic cavity and lined by high-pressure intercostal arteries. More than 80 % pleural flow is reabsorbed through the visceral pleura covering the lungs and the rest by subserosal lymphatics. Malignant pleural effusion develops when there is irritation of pleural serosa which produces more fluid and reabsorbs less. It may also occur when there is obstruction of the lymphatics or venous obstructions. Furthermore, when there is a large amount of free tumor cells in the pleural fluid along with increased intrathoracic protein, pleural effusion occurs.
Malignant cytology is found in up to 50 % of all patients with pleural effusion. While positive cytology is an indication of intrathoracic therapy, the status of exudates is another indication, as determined by pleural fluid LDH level > 200 mg/dl, pleural/serum LDH ratio > 0.6, or pleural/serum protein > 0.5. Cancer patients with pleural fluid consisting of transudate do not respond well to intrathoracic therapy and should be treated with systemic therapy. For intrathoracic therapy, pleural biopsy may be the last resort to establish malignant pleural effusion by tumors to differential from other etiologies including inflammation (e.g., sarcoidosis and systemic lupus) and infection (e.g., tuberculosis).
Intrathoracic therapy is typically performed by thoracostomy and may involve sclerosing agents to obliterate the peritoneal cavity through chemical inflammation. Tetracycline is usually the initial choice, followed by cytocidal and inflammatory agent’s quinacrine, nitrogen mustard, bleomycin, or 5-FU, with response rate of 60–80 %. There has been no comparative study between sclerosing and inflammatory agents with radionuclide therapy of malignant pleural effusion. Radionuclide therapy is usually the last resort, partly because of expenses and partly because of radiation concerns. The use of Au-198 colloid was able to illicit 50–75 % total or partial response in malignant pleural effusion but was subject to long-term radiation hazard consideration and is no longer commercially available (Mohlen and Beller 1979; Croll and Brady 1979; Leff et al. 1978; Fleay 1971; Sklaroff 1965; Botsford 1964; Dybicki et al. 1959; Lehman 1954; Colby 1954). Since early reports from the 1950s, P-32 chromic phosphate suspension has been applied to treat malignant pleural effusion with up to 50 % responses. Typically, a thoracentesis is performed, initially followed by 2–3 mCi Tc-99 m sulfur colloid to confirm loculation of injectate. After satisfactory distribution is established, 10–15 mCi of P-32 chromic phosphate suspension is injected and flushed by 50 ml of saline. The patient is to change position every 10 min for 2 h to facilitate distribution within the pleural space. This is a palliative procedure and has no impact on survival. The rate of pleural fluid production or the frequency of thoracentesis required for relief symptoms is used as the response (Sklaroff 1965; Botsford 1964; Scheer 1964; Shah and Warawdekar 1964; Reeve and Myhill 1962; King et al. 1952). The mechanisms of P-32 are nonspecific ablation of freely floating tumor cells and pleural serosa and inflammation of pleura. Under similar mechanisms, an earlier trial was reported in 1956 using 9.5–30 mCi of Y-90 mixed with 100–200 mg of nonradioactive yttrium trichloride to nonspecifically ablate pleural effusion resulting in 76 % response. The authors claimed advantages of shorter physical half-life of 61 h and ready detoxification schemes using EDTA but this approach has not been repeated (Scheer 1964, 1965). Radiolabeled tumor-targeting monoclonal antibodies (mouse I-131 HMFG1, HMFG2, and AUA1) were used in a trial and reported response in 9/11 patients. This approach was also applied to treat malignant pericardial effusion (Kalofonos et al. 1994; Kosmas et al. 1990; Malamitsi et al. 1988; Pectasides et al. 1986). While the advantage of tumor-targeting versus nonspecific ablation in this latter approach needs to be proved, the disadvantage of mouse antibodies also needs to be addressed for more extensive application.
Malignant pericardial effusion occurs with cancers from the breast and lungs, lymphomas, melanoma, and sarcomas. A small amount of fluid is normally found in the pericardial sac and is typically drained by lymphatics. With tumor seeding, irritation of serosa, production of fluids, and obstruction of lymphatics, there will be progressive swelling, to the degree of cardiac tamponade, dysrhythmia, and decreased cardiac output. The diagnosis can be made by cytology through pericardiocentesis, which is typically done in the subxiphoid approach. The goal of the treatment is to relieve symptoms of cardiac tamponade and to reduce the elevated risk of sudden death. Treatment modalities include external beam therapy up to 15 Gy over 10 days as well as instillation of chemotherapeutics, sclerosing agents, or radiopharmaceuticals similar to the treatment of malignant effusion. Radionuclide therapy may indeed be preferable when a patient develops pericardial effusion under chemotherapy. Prior radiation therapy to the chest is not a contraindication to radionuclide therapy. Typically, after pericardiocentesis using a peritoneal catheter under ultrasound guidance, 0.5 mCi of Tc-99 m DTPA in 25 ml is instilled to evaluate pericardial distribution. Upon confirmation of distribution inside the pericardial space, 5 mCi of P-32 chromic phosphate in 30–50 ml is instilled followed by immediate removal of the catheter. An earlier report indicated hemopericardium as a potential complication (Blau et al. 1977). Two studies using P-21 chromic phosphate reported high response rates as much as 16/28 patients with little complications (Martini et al. 1977; Covington and Hilaris 1973). Some contribute the high response rates to more complete removal of effusion fluid before instillation of radiopharmaceuticals. Another study report responses in 3/3 patients using mouse monoclonal antibodies HMFG1, HMFG2, and AUA1 which are labeled with I-131 (Pectasides et al. 1986) but the advantages of tumor targeting are still to be realized.
2.3 Peritoneal Carcinomatosis
Malignant ascites occurs as results of increased production of peritoneal fluid because of peritoneal irritation and/or reduced removal of peritoneal fluid from obstruction of lymphatics. It may be exacerbated by low plasma oncotic pressure. The most common type of malignant ascites is from solid tumor implants from exfoliation of tumor cells with extensive implants on viscera. Tumor cell counts in peritoneal fluid range from 50–1000 cells/microliter. Intracavitary radionuclide therapy may be able to treat free flowing tumor cells and to induce fibrosis of the serosa but is rarely effective in controlling visceral metastases because of limited ranges of emissions. A less common type arises from obstruction of venous return or lymphatics by large tumor mass. Peritoneal fluid tumor cell count is low and response to intracavitary therapy is little. Another type of malignant ascites involves high tumor cell counts to over 4000 cells/micro liter and responds best to intracavitary radionuclide therapy.
While there are other causes of ascites such as congestive heart failure, cirrhosis, pancreatitis or peritonitis from infection, malignant ascites occurs most often in metastatic ovarian, renal, gastrointestinal cancer and occasionally with mesothelioma or lymphoma. The US FDA restricts intracavitary radiocolloid therapy to ascites caused by metastases. While demonstration of malignant cells is not required, exclusion of other treatable nonmalignant etiologies is prudent and critical for good response. Intracavitary radionuclide therapy is indicated for palliation when systemic therapy and parathecenthesis fail to relieve symptoms of abdominal distention, dyspnea, cough, or hiccoughs from diaphragmatic irritation.
For the administration of radionuclide, typically a fenestrated peritoneal dialysis catheter is inserted under aseptic condition under image guidance and the catheter is secured to the surface of the abdomen. After ascites fluid is removed, a tracer dose of 1 mCi of Tc-99 m sulfur colloid is injected and flushed with 250 mL saline. The patient will be asked to roll from side to side to distribute the tracer before scintigraphy is performed to confirm distribution to the peritoneal cavity. Then, 15–25 mCi of P-32 chromic phosphate in 500 mL of saline is injected into the catheter before it is removed. The patient will then be positioned to lying on the left side, the right side, inclined with head down, and inclined with feet down for 5 min each and bremsstrahlung imaging may be performed to confirm distribution. P-32 colloids are rapidly cleared from the peritoneal fluid with only 5 % retention in 24 h and the remainder plating out over the serosal surface. Radiocolloids are also cleared by macrophages and diaphragmatic lymphatics (Wilkinson 1996). The potential adverse effects are nausea, vomiting, diarrhea, and abdominal pain, all related to radiation peritonitis. Up to 9 % patients may develop small bowel obstruction with concurrent external beam radiation (Tharp and Hornback 1994).
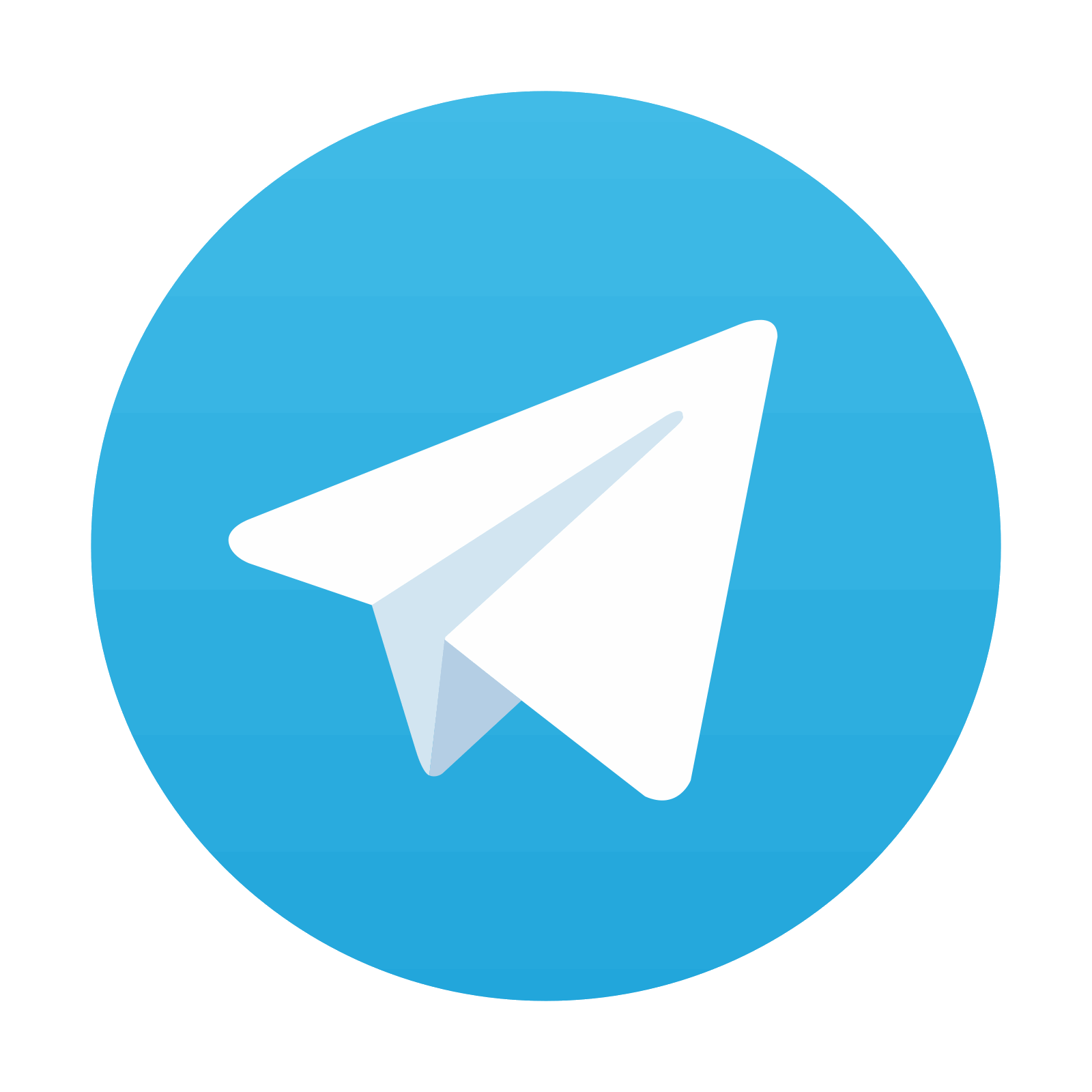
Stay updated, free articles. Join our Telegram channel
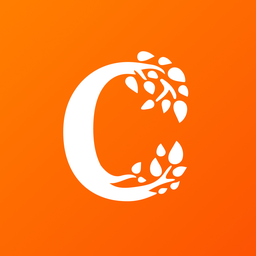
Full access? Get Clinical Tree
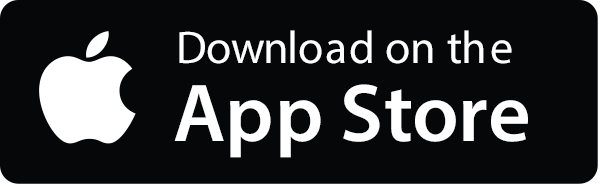
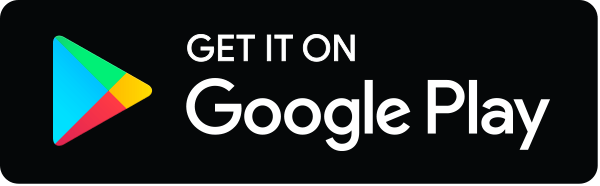