Tumor/location
Radiotherapy indications (by rising stage)a
Other components of multimodal therapy
Aim of treatment
Main imaging modality usedb
Glioma
Adjuvant RT/RCT
Resection
Cure
MRI
Definitive RT/RCT
Chemotherapy
Brain metastases
Radiosurgery
Resection
Local control
MRI
SFRT
Chemotherapy
Head and neck cancer
Definitive RT
Resection
Cure
MRI
Adjuvant RT/RCT
Chemotherapy
CT
Definitive RCT
FDG-PET
Breast cancer
Adjuvant RT
Resection
Cure
Mammography
Endocrine therapy
CT
Chemotherapy
Lung cancer
SBRT
Resection
Cure
CT
Neoadjuvant RT
Chemotherapy
FDG-PET
Adjuvant RT
Definitive RCT
Lung metastases
SBRT
Resection
Local control
CT
Chemotherapy
FDG-PET
Esophagus carcinoma
Definitive RT (brachytherapy)
Resection
Cure
CT
Neoadjuvant RCT
Chemotherapy
FDG-PET
Definitive RCT
Liver metastases
SBRT
Resection
Local control
MRI
Chemotherapy
Rectal cancer
Adjuvant RT
Resection
Cure
CT
Neoadjuvant RT
Chemotherapy
MRI
Prostate cancer
Definitive RT
Resection
Cure
CT
Adjuvant RT
Endocrine therapy
MRI
RT of recurrence
Gynecologic tumors
Definitive RT
Resection
Cure
CT
Adjuvant RCT
Chemotherapy
MRI
Definitive RCT (all including brachytherapy)
The technical progress of recent years has been made possible by advancing computational resources leading to improved planning and application technologies and by congenial engineering developments like multi-leaf collimators and on-site positioning control. However, all these developments would not have been possible without medical imaging (Fig. 4.1). By the 1980s, CT was integrated into radiotherapy planning [1]. While the first intentions were rather aimed at better geometrical and physical treatment planning (until today, CT tissue density data are used for the calculation of beam attenuation within the patient), it became obvious very soon that the (patho)anatomical informations could also be used for target definition and normal tissue identification. This has triggered the development of modern target volume concepts and planning techniques. Today, we have highly precise technologies of treatment planning an delivery available, e.g., intensity-modulated radiation therapy (IMRT) and stereotactic radiotherapy (SRT, SBRT), which enable safe high-dose treatment of precisely defined target volumes with simultaneous sparing of normal tissues [2]. IMRT techniques enable the radiation oncologist to spare organs like the spinal cord or the parotid glands while giving therapeutic radiation doses to neighboring tumors, e.g., in the head and neck area [3]. It is possible to spare the rectum and the bladder while giving high-dose curative treatment to prostate cancer [4] and to cure benign tumors of the base of the skull with a minimum of neurological late sequelae [5]. Stereotactic radiotherapy of brain metastases preserves the quality of life of many of cancer patients without neuropsychological toxicity [6, 7], while stereotactic radiotherapy of localized lung cancers in inoperable patients has improved the prognosis of this patient group on an epidemiologically measurable scale [8].
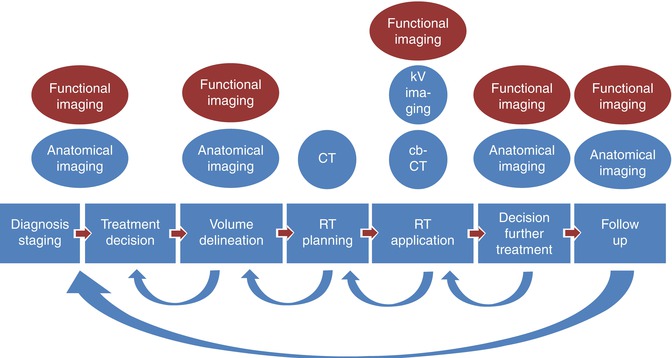
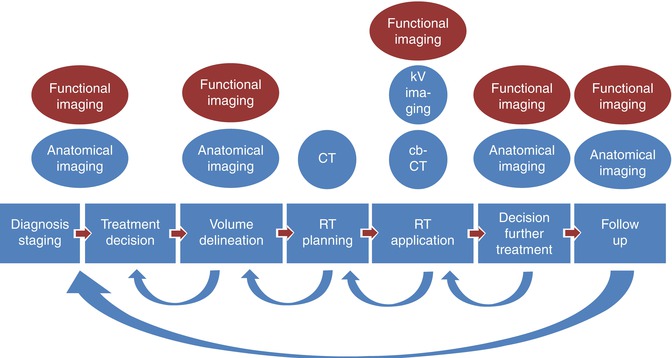
Fig. 4.1
Role of imaging in the radiotherapy process: the arrows showing the various feedback slopes triggered by often imaging based information
Modern IMRT techniques have also opened the door to the application of various dose levels within one treatment. Practical implementations are simultaneously integrated boost techniques, where, e.g., the macroscopic tumor and the areas of assumed microscopic spread are covered by high- and intermediate-dose ranges (Fig. 4.2) [9]. Beyond this, the so-called dose painting has become possible, where radiotherapy adaptation within a given target can be defined by biological properties of certain sub-volumes. Using IMRT, e.g., hypoxic regions within a tumor assumed to be radioresistant, might be treated with dose prescriptions on the basis of hypoxia-PET imaging [10]. This is a very interesting field for the use of molecular imaging techniques for radiotherapy. First studies in the head and neck tumors presently address this option [11]; however, clinical outcome data have to be awaited before introducing these techniques into clinical practice.
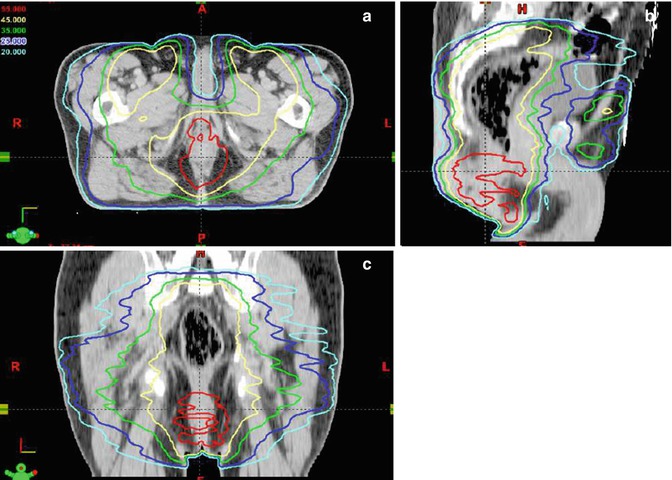
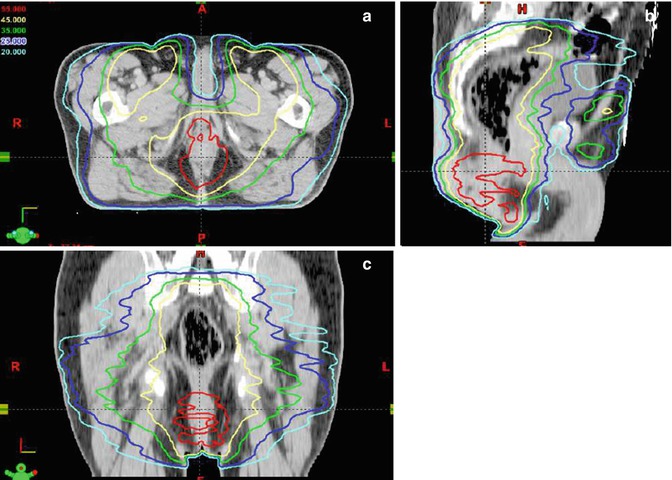
Fig. 4.2
IMRT-based simultaneous integrated boost technique: three-plane view (a, b, c) of an RT-treatment plan for the curative simultaneous radiochemotherapy of a patient with anal cancer. 54 Gy are given to the primary tumor and 45 Gy to the nodal CTV. Note the high dose prescribed to the primary tumor (red isodoses) is given simultaneously to the medium-dose treatment to the adjuvant nodal areas (yellow isodoses)
The new particle beam technologies like proton and heavy ion therapies promise even better dose distributions. Due to their physical properties, the treatment dose can be delivered to a very confined area with perfect sparing especially of the normal tissues behind the target. However, being very sensitive to the physical properties of the tissue, these methods also rely on imaging as a prerequisite for successful treatment planning and delivery [12].
Re-irradiation has been largely avoided in the past, due to the risk of severe normal tissue toxicity. But with a more precise definition and 3D dose documentation together with growing clinical and radiobiological knowledge, re-irradiation can now also be given more frequently, provided that restaging reveals localized tumor progression and the area of progression can be defined clearly (Fig. 4.3) [13–17].
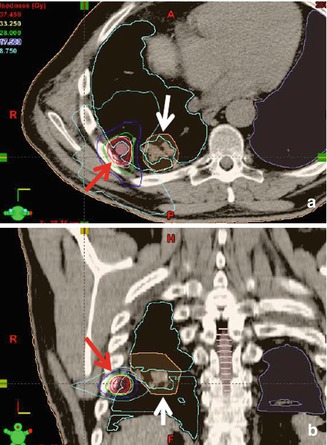
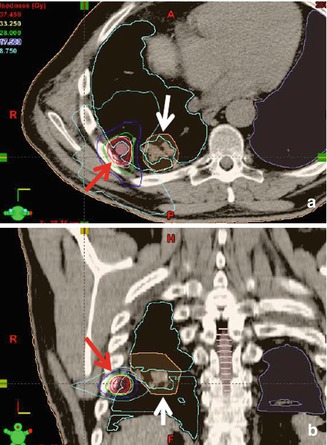
Fig. 4.3
Re-irradiation of a patient with metachronous pulmonary metastases of colon cancer in axial (a) and coronal (b) plane. The actual target volume of IMRT-SBRT to a metastasis near the thoracic wall (red arrow) lies in direct neighborhood of the former treatment volume (white arrow) now showing post-RT fibrosis. The IMRT technique enables the avoidance of the former high-dose area (light blue isodose)
Overall, in the light of all these new possibilities, accurate staging and depiction of the geometry of malignant spread are a prerequisite for effective and precise radiotherapy applications. While very high doses are given inside of target volumes, a rather low exposure is often achieved in their direct neighborhood (Fig. 4.4). Technical and interpretational imaging errors may directly translate to impaired local tumor control and/or increased risk for normal tissue toxicity. Furthermore, erroneous interpretation of posttreatment changes may lead to unnecessary interventions and patient discomfort.
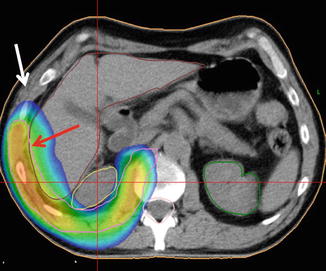
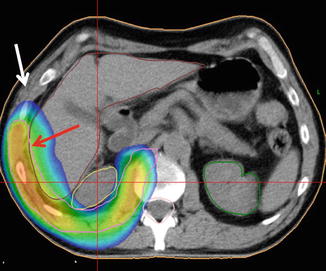
Fig. 4.4
IMRT plan of a patient with pleural mesothelioma. Note that high-dose areas (red arrow) are very confined to the PTV (pink contour), while the neighboring thoracic wall (white arrow) and the right kidney (yellow contour) receive far lower doses. In case of erroneous target volume delineation, the risk for a local recurrence in the white arrow area would be high
This chapter will go through the relevant applications of imaging in radiotherapy and aims at increasing the awareness of the reader about radiotherapy specific questions and requirements for molecular imaging.
4.2 Diagnosis/Staging
Imaging is mandatory in the process of diagnosis and staging of tumors, as this is a prerequisite for interdisciplinary guideline-based treatment decisions. The decision for the appropriate radiotherapy concept cannot be made without adequate imaging. Obviously, a local aggressive treatment with curative option like stereotactic radiotherapy will only be of benefit for the patient in a metastasis-free situation. During initial evaluation of the benefit of improved staging, several groups reported that the detection of unexpected metastases by FDG-PET often led to a palliative rather than a curative concept with less unnecessary interventions in incurable situations [18–22]. However, the early accurate diagnosis of patients in an oligo-metastatic situation has also led to the discussion of new locally curative treatment concepts for this population, and first data on long-term survivors have been published [23]. The advances in molecular imaging, e.g., the use of FDG-PET/CT for solid tumors, have therefore significantly improved patient care (Fig. 4.5). It has been shown, that the mere fact, to have performed a FDG PET during the staging of a cancer patient can be a prognostic factor by itself [24].
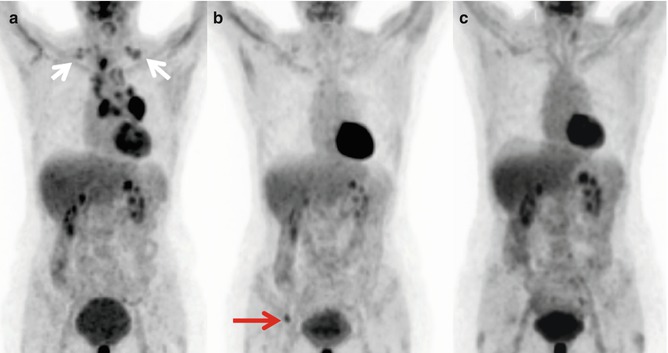
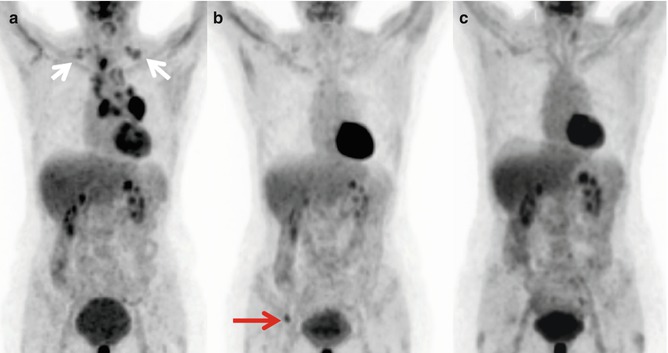
Fig. 4.5
Serial FDG-PET-CT whole body scans (MIPs) showing long-term survival after FDG-PET-based treatment of a 50-year-old woman with non-small cell lung cancer. (a) Initial situation with T3 N3 M0 NSCLC (supraclavicular spread, white arrows) before combined radiochemotherapy. (b) Complete metabolic remission of the thoracic tumor 9 months later with diagnosis of a solitary bone metastasis (red arrow). (c) Complete metabolic remission 15 months after radiotherapy of the metastasis and bisphosphonate treatment. The patient is alive without recurrence 3 years after initial presentation
Although histology is a prerequisite for oncologic decision making, there may be certain situations in which the decision for radiotherapy can be based on imaging alone, e.g., repeated chest CT + FDG-PET/CT in medically inoperable patients with small lung cancers. Although controversially discussed, it has been shown that missing histology in cases with clear imaging based diagnosis is prognostically irrelevant [25].
In contrast, further information derived from imaging may be of prognostic significance. Beyond TNM staging information, data derived from molecular imaging like SUVmax, metabolic volume [26], imaging assessed tumor hypoxia [27], perfusion parameters and MRI-spectroscopic parameters [28], have been shown to predict outcome. Like prognostic indices based on pretreatment data (lymphoma, breast cancer scores), these factors might be used to stratify treatments in the future. However, such concepts will need to be tested in clinical trials before general use.
In this context, it is important to know that the timing of imaging for radiotherapy needs to be discussed together with the initial treatment decision. In many cases, radiotherapy treatment volumes will relate to initial tumor spread, irrespective of whether radiotherapy will be given as initial treatment or in a later, e.g., adjuvant, situation or after (induction) chemotherapy. Consequently the radiotherapy portals may need to include all pretreatment tumor spread. As systemic treatment may lead to early decrease of signals from molecular imaging, the lack of a pretreatment scan may cause an undertreatment of the patient [29]. On the other hand, tumor progression may be present between initial staging and onset of radiotherapy. Hence, if imaging is to be used for the planning of primary radiotherapy or radiochemotherapy, it may be necessary to repeat scans. This has been shown impressively by the Melbourne group, who reported on a 32 % risk of progression in FDG-PET within 24 days after initial staging of locally advanced lung cancer [30].
After a decision for radiotherapy has been made, the specific interest of the radiation oncologist for further development of his treatment concept lies in the geometry of the disease. Firstly, the local situation of the primary tumor is highly relevant for the choice of radiotherapy techniques and achievable doses. Here, molecular imaging, e.g., amino acid PET may help in giving the correct topography of brain tumors [31] or FDG-PET in the delineation of primary or recurrent head and neck tumors [32].
Secondly, nodal staging is a crucial topic for radiotherapy concepts of many solid tumors. In the primary radiotherapy and/or radiochemotherapy of most cases with head and neck tumors, lung and esophageal cancer, upper abdominal malignancies, and pelvic tumors, a certain radiation dose will be given to macroscopically unaffected nodal areas, and this adjuvant radiotherapy of nodal regions is a main contributor for treatment toxicity. Obviously, the diagnosis of macroscopic nodal involvement will influence this treatment decision. Furthermore, the accuracy of the staging method used may influence the target of radiotherapy. A prominent example is the omission of large mediastinal target volumes in the primary radiochemotherapy for lung cancer after the advent of FDG-PET [33
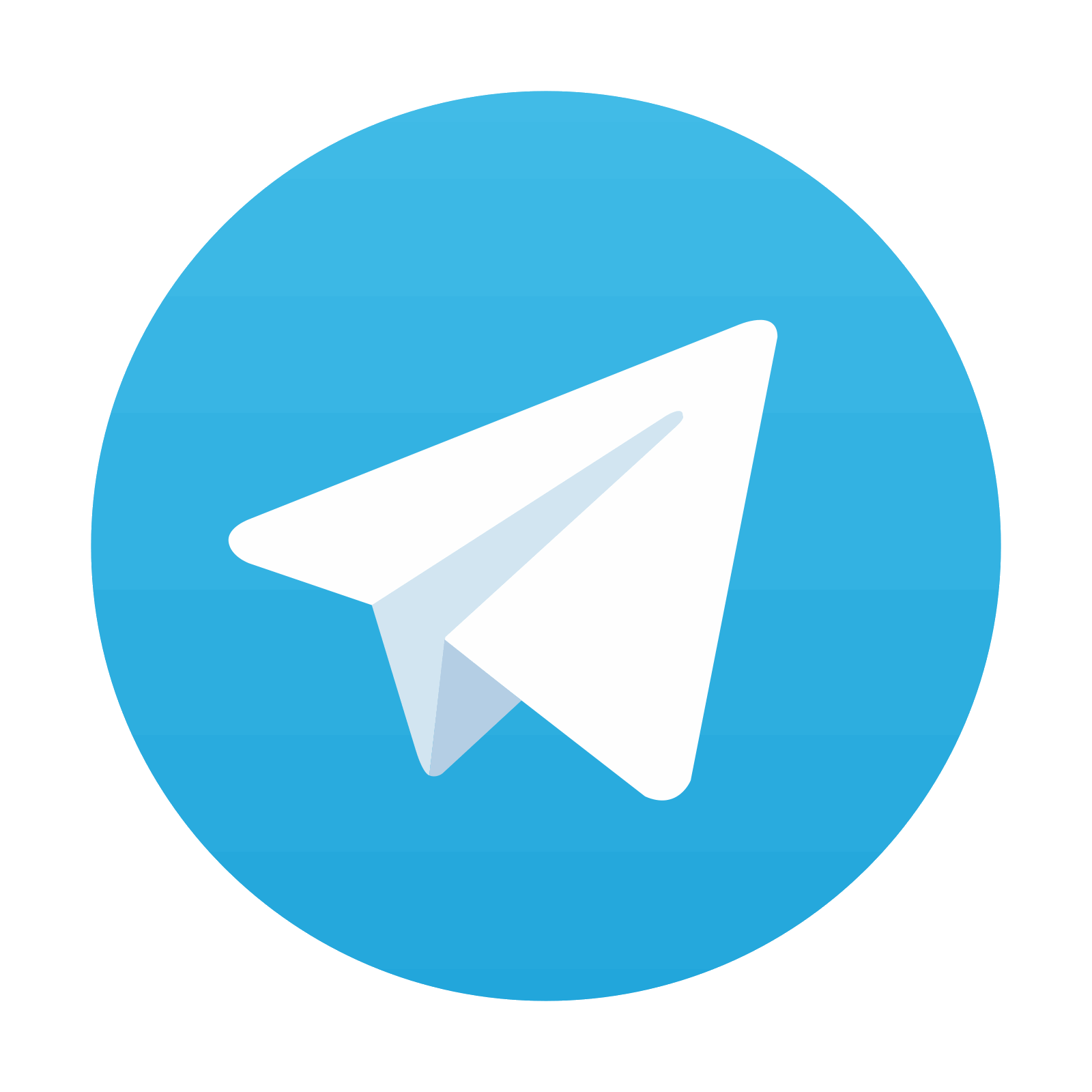
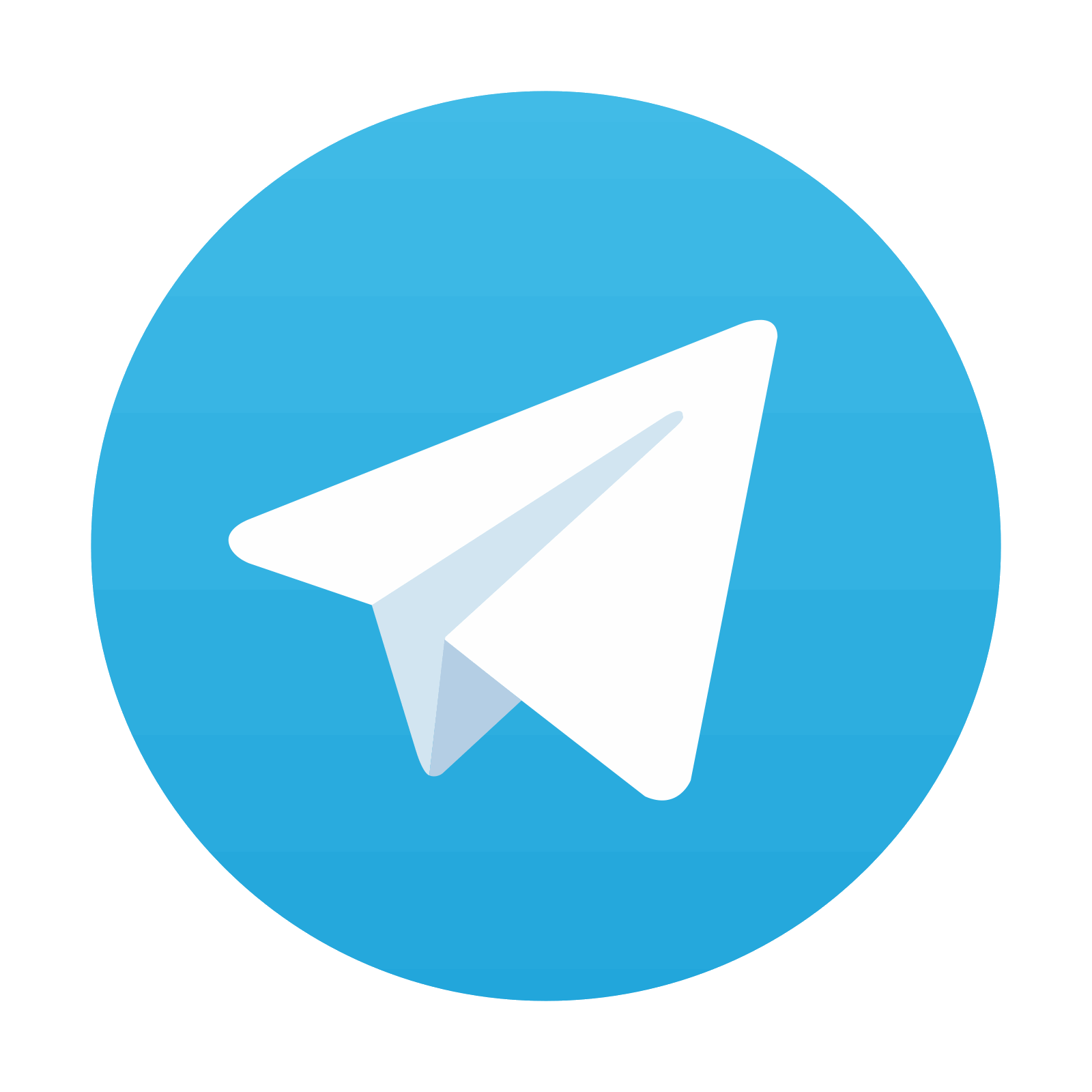
Stay updated, free articles. Join our Telegram channel
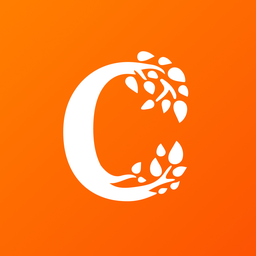
Full access? Get Clinical Tree
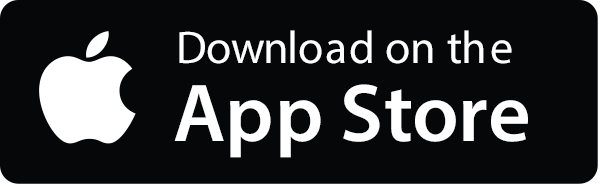
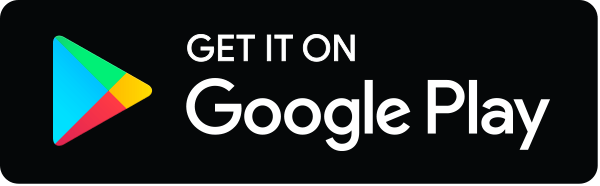