Cervical spine
Vertebral arteries
Deep and ascending cervical arteries
Thoracic and upper lumbar spine
Subclavian arteries via deep cervical artery
T1 and T2 segmental/intercostal arteries
Aorta
Thoracic segmental arteries (intercostal)
One pair of superior/supreme intercostal artery (above T3)
One pair at each vertebral level from T3–T12
Lumbar segmental arteries
One pair at each vertebral level from L1–L4
Lower lumbar spine and sacrum
Internal iliac artery (iliolumbar and lateral sacral arteries)
Median sacral artery (branch of the aorta)
It is important to remember that the segmental arteries are named by the level they supply and not by the level from which they originate. Segmental arteries supply all of the dorsolateral tissues of a single metamere, except the spinal cord. There are extensive anastomoses between segmental arteries with important connections both above and below a given level, as well as contralaterally. The segmental arteries divide into three major trunks: (i) lateral or ventral (posterior intercostal or lumbar artery), (ii) middle or dorsal (muscular and cutaneous branches), and (iii) medial or spinal [13].
Radicular Arteries
Radicular artery along with the anterior and posterior spinal arteries branches out from the medial (spinal) trunk, after entering the spinal canal at intervertebral foramen. Further at each nerve root level, the radicular artery ramifies [14] into:
1.
Radiculoradial artery – supplies both the dura and the nerve roots.
2.
Radiculomeningeal artery – supplies only the dura.
3.
Radiculomedullary artery – supplies the spinal cord. Unlike other two branches, radiculomedullary branches are present only at some nerve root levels.
Arterial Supply of Spinal Cord
1.
Superficial or external arterial system
2.
Deep or intrinsic arterial system
Superficial Arterial System of the Spinal Cord
At the surface of the cord, two arterial systems can be described (Fig. 1):
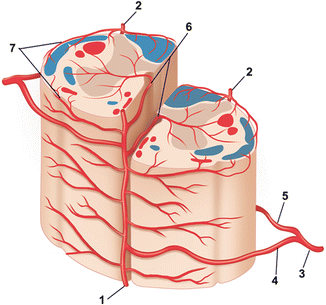
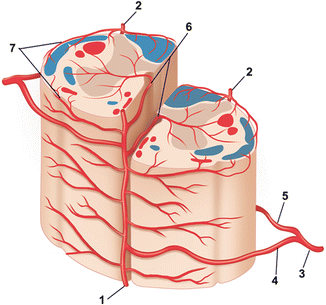
Fig. 1
Arterial supply of the spinal cord: (1) anterior spinal artery, (2) posterior spinal artery, (3) spinal trunk of segmental artery, (4) anterior radiculomedullary artery, (5) posterior radiculomedullary artery, (6) central (sulcal) arteries, (7) vasocorona
1.
Longitudinal arterial trunks: extend along the long axis of the spinal cord and are constituted by one anterior spinal artery and two posterior (or posterolateral) spinal arteries, which receive flow from the anterior and posterior radiculomedullary arteries, respectively
2.
Pial plexus: covers the periphery of the spinal cord
Longitudinal Arterial Trunks
Anterior Spinal Artery
The anterior spinal artery (ASA) is typically formed at the level of the foramen magnum by the confluence of descending branches of the intracranial segments of the vertebral arteries (above the posterior inferior cerebellar artery (PICA)). The descending branches from both vertebral arteries may join at the C2–C4 level [15] and travel along the anterior sulcus of the spinal cord to the conus medullaris. ASA has variable caliber (diameter 0.2–0.8 mm); it is thinnest in the thoracic cord and thickest in the region of the conus medullaris [15]. The ASA supplies the anterior two-thirds of the spinal cord tissue (including the anterior horns and the spinothalamic and corticospinal tracts) by central and pial branches.
The ASA is usually continuous, and during its long course, it receives additional arterial supply via the anterior radiculomedullary arteries in order to maintain adequate blood flow to the entire spinal cord. As a result, the ASA should not be thought of as a single straight artery but rather as a consecutive series of anastomotic vascular loops [16].
ASA receives major contribution from the radiculomedullary arteries at three regions: cervicothoracic, mid-thoracic, and thoracolumbar, with watershed areas at the border of each region, especially in the upper thoracic region. The most important anterior radiculomedullary arteries and most easily recognized in angiography are the artery of cervical enlargement in the cervical and upper thoracic region and artery radiculomedullaris magna, also known as the artery of Adamkiewicz (AKA) in the lower thoracic and lumbar region (Fig. 2).
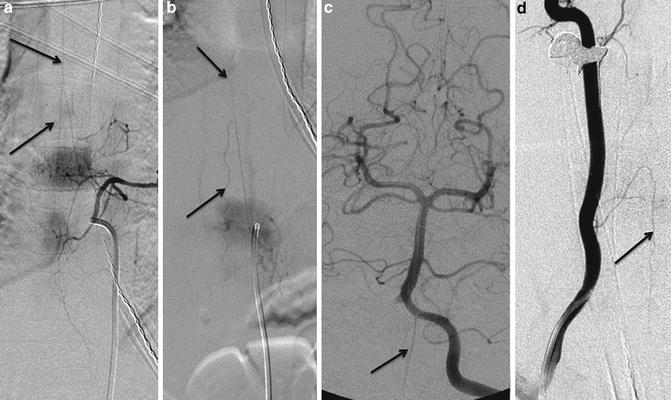
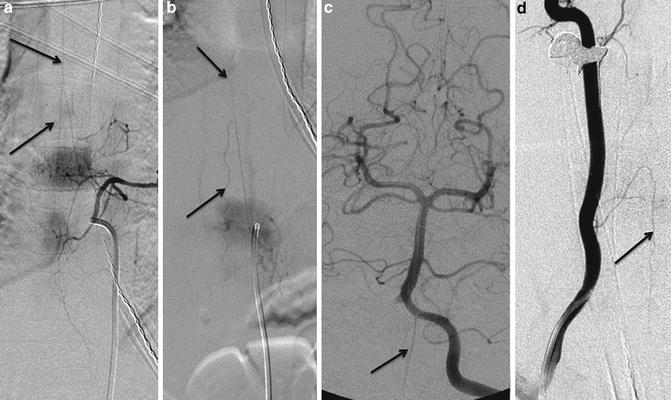
Fig. 2
Anterior spinal artery: DSA demonstrating the midline anterior spinal artery (ASA) with contribution from the dominant radiculomedullary branch in the thoracolumbar segment, artery of Adamkiewicz with typical hairpin appearance (a, b). Note the vertebral artery contribution to ASA in the cervical cord segment (c, d)
The mid-thoracic spinal cord is more vulnerable to ischemic compromise as there is minimal collateral supply to the spinal cord superior to the junction of the AKA with the ASA. The ASA continues caudally past the conus as an insignificant branch to the filum terminale; the more important, functional continuations of the ASA are the “rami cruciantes” that surround the conus and provide robust “basket” anastomoses with the posterior spinal arteries [17].
Posterior Spinal Arteries
The two posterior spinal arteries (diameter <0.5 mm) also originate at the level of the foramen magnum by branches of the ipsilateral vertebral or posterior inferior cerebellar arteries and travel along the posterolateral surface of the spinal cord, receiving supply at various levels from the posterior radiculomedullary arteries (Fig. 3). 11–16 feeders contribute to the posterior spinal artery (PSA) at various levels through the spine. The largest posterior radiculomedullary artery often enters below the level of the AKA [15].
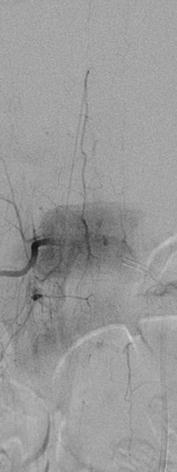
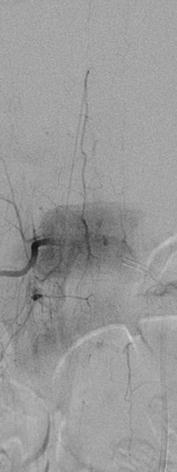
Fig. 3
Posterior spinal artery: DSA demonstrating the posterior spinal artery (PSA) in the lower thoracic cord. Note the paramedian location of the PSA compared to ASA and the sharper angle of the hairpin curve
Throughout its course, each PSA gives off branches that supply the posterior third of the spinal cord, including the posterior columns, dorsal gray matter, and superficial dorsal aspect of the lateral columns of the spinal cord.
Radiculomedullary Arteries
At several levels, the radicular artery gives branches that follow the anterior and/or posterior nerve roots to supply the spinal cord, called the radiculomedullary arteries. The average number of anterior radiculomedullary arteries is 6 (range 2–14) [18, 19], whereas the number of posterior radiculomedullary arteries varies from 11 to 16 [15].
Radiculomedullary Artery of Cervical Enlargement
There are 2–3 anterior radiculomedullary arteries in the cervical region. The most important supply to the cervical ASA is usually located between the C4–C8 levels of the spinal cord and is termed the artery of cervical enlargement [20], often a branch of the deep cervical artery, and usually accompanies the C6 nerve root.
Artery of Adamkiewicz (AKA)
The most important anterior radiculomedullary artery and the one most easily recognized in angiography is the artery radiculomedullaris magna, also known as the artery of Adamkiewicz (AKA). It has a diameter of 0.5–1.0 mm [15] and almost always arises in the thoracolumbar region, between T8 and L2 in 75 % of cases [16, 21–23]. It has, however, been identified as superiorly as T5 and as inferiorly as L4 [19]. In 80 % of cases, it is found in the left side. The AKA forms the classic “hairpin” loop when it reaches the ASA and gives off a thin ascending branch and a larger descending branch.
Pial Plexus
Besides the direct connections between the ASA and PSAs at the conus medullaris, there is an extensive arterial network on the entire surface of the spinal cord formed by effective anastomoses between the transverse and oblique branches from the ASAs and PSAs. This superficial rich anastomotic network is termed the pial plexus (vasocorona) and has been visualized on microangiograms of the spinal cord [15]. This network is responsible for vascularizing the periphery of the spinal cord.
Intrinsic Arterial System
The spinal cord parenchyma is supplied by the intrinsic arterial system, which is subdivided into a central (centrifugal) system and a peripheral (centripetal or vasocorona) system [13]. The central system is comprised of the central arteries (sulcal or sulcocommisural arteries; diameter 0.06–0.40 mm), which originate from the ASAs and travel into the anterior median fissure, penetrate into the cord either on the left or right, and branch centrifugally, mainly within the gray matter [19]. The peripheral system (vasocorona; diameter 0.1–0.2 mm) [19] consists of small perforators (rami perforantes) that originate from the pial plexus at the periphery of the spinal cord and course into the white matter centripetally.
Watershed Territories of the Spinal Cord
The concept of watershed areas has been applied to the areas of relative poor vascularity in the rich spinal cord vascular network. As detailed in the anatomy section, the spinal cord has rich vascularity contributed from the longitudinal and circumferential networks. The blood supply of the cord thus depends on the integrity of half a dozen radicular arteries so far as the anterior three-quarters of the cord is concerned and on a slightly greater number of posterior radicular arteries for the nourishment of the posterior third.
Given the relative paucity of these networks in certain regions, there exists “watershed territories” in both the networks.
Longitudinal Anastomosis
Although radicular arteries accompany the spinal nerves at each level, the radiculomedullary branches supplying the spinal cord are present only at few levels. The average number of anterior radiculomedullary arteries is 6 (range 2–14) [18, 19], whereas the number of posterior radiculomedullary arteries varies from 11 to 16 [15]. The superior cord segment involving cervical and upper thoracic cord (up to T2/T3) is usually supplied by vertebral arteries and 2–3 anterior radiculomedullary arteries, including the artery of cervical enlargement [20], often a branch of the deep cervical artery, and usually accompanies the C6 nerve root. Similarly 2–3 anterior radiculomedullary arteries, including the artery of Adamkiewicz (AKA), usually supply the inferior cord segment involving the lower thoracic and lumbar cord. The intermediate cord segment involving the mid-thoracic cord (T4–T8) is a poorly vascularized segment with paucity of the radicular arteries, although occasional radicular artery is present at T7 level. In addition to the overlapping areas between the upper, mid, and lower cord segments, the entire mid-thoracic cord due to its paucity of the radiculomedullary arteries is considered as an anatomical “watershed territory” of the spinal cord.
Circumferential Anastomosis
Within the intrinsic arterial system, there is anastomosis between the central (centrifugal) system and the peripheral (centripetal or vasocorona) system [13]. The sulcal artery is a branch of the anterior spinal artery, which, centrally oriented, runs through the anterior median sulcus, enters the cord, and supplies most of the gray matter and the adjacent white matter. The peripheral system (vasocorona) [19] consists of small perforators (rami perforantes) that originate from the pial plexus at the periphery of the spinal cord and course into the white matter centripetally. Located in between, there exists a border zone that is supplied by both the central and peripheral arteries.
Ischemic Vulnerability of the Spinal Cord
Historically, the literature has supported the notion of a spinal cord “watershed zone” of ischemic vulnerability centered at the mid-thoracic level (T4–T8 level) [24, 25]. However, Duggal et al. showed that >95 % of cardiac arrest and hypotensive patients with associated ischemic myelopathy had predominant involvement of the lumbosacral level followed by cervical level with relative sparing of thoracic levels. None of the examined patients in their study had neuronal necrosis limited to the thoracic level only. Authors concluded that, although the thoracic spinal cord may be the anatomic watershed zone with respect to regional blood supply, the lumbosacral cord neurons appear to be more susceptible to ischemia.
Neuronal necrosis restricted to the lumbosacral level of the spinal cord was first reported by Gilles and Nag [26] in 6 neonates suffering transient cardiopulmonary arrest. These findings were later confirmed in a larger series by Azzarelli and Roessmann [27] in their material from 16 patients, including 11 adults with ischemic myelopathy, which demonstrated lesions located throughout the spinal cord in nine cases and restricted only to the lumbosacral cord in four patients.
High metabolic demands and the large number of neurons in the gray matter make it more vulnerable to ischemia than the white matter [24]. The spinal cord blood flow analysis in primates showed a higher blood supply to the gray matter (57.6 +/− 2.3 ml/100 g/min), due to the much higher metabolic rate compared to the white matter (10.3 +/− 0.2 ml/100 g/min) [24]. Similarly primate studies’ total flows in cervical, upper thoracic, and lumbar areas were 14.9 + 1.4, 10.4 ± 0.8, and 19.7 ± 1.2 ml/min/100 g, respectively [24]. The lowest total flow is observed in the thoracic cord; in part, this difference has been attributed to the relative decrease in the gray matter at this level.
Etiology
Spinal cord infarction (SCI) can be categorized into three groups (Table 2):
Table 2
Etiology of spinal cord infarction
Ischemic infarction |
Hypoperfusion |
Aortic surgery |
Aortic dissection |
Systemic hypotension |
Thrombosis of abdominal aortic aneurysm |
Atherosclerosis of segmental arteries |
Vertebral artery dissection |
Mechanical injury (surfer’s myelopathy) |
Abdominal/pelvic surgeries |
Spinal surgery or intervention |
Embolism |
Thromboembolism |
Cardiac arrhythmias |
Valvular heart disease |
Therapeutic embolization (spinal, renal, and bronchial) |
Aortic catheterization |
Fibrocartilaginous embolism |
Septic (infection) embolus |
Hemorrhagic infarction |
Subarachnoid hemorrhage |
Arterial dissection |
Ruptured spinal aneurysm |
Dural arteriovenous fistula (DAVF) |
Perimedullary arteriovenous malformation (AVM) |
Anticoagulation |
Bleeding diathesis |
Epidural/subdural hemorrhage |
Spontaneous rupture of spinal DAVF, perimedullary AVM [29] |
Intramedullary hemorrhage – hematomyelia |
Spinal angiomas [29] |
Ruptured spinal AVM |
Cavernoma |
Spinal tumors |
Venous infarction |
Spinal DAVF, perimedullary AVM |
Cord compression from extramedullary lesions |
Tumor (e.g., pancreatic) embolus [30] |
Decompression sickness (air embolus) |
Miscellaneous |
Substance abuse (e.g., cocaine) [34] |
Drug induced (e.g., sildenafil citrate) [35] |
1.
Ischemic infarction
2.
Hemorrhagic infarction
3.
Venous infarction
Ischemic Spinal Cord Infarction
This is the most common type of spinal cord infarction. The common etiological factors in this group are included in two distinct groups: hypoperfusion and embolism. The individual risk factors are discussed in detail below:
Hypoperfusion Related
Aortic Surgery
Paraplegia as a complication of aortic surgery in humans was first reported in 1956 by Adams et al. [36]. Surgical repair of thoracic and thoracoabdominal aortic aneurysms and aortic dissections (Fig. 4) are the most common etiology for SCI [7, 9, 10]. Although this complication is reported both after open and endovascular repair of ruptured aneurysm, its incidence is lower by using endovascular techniques, partly related to the patient selection bias [37].
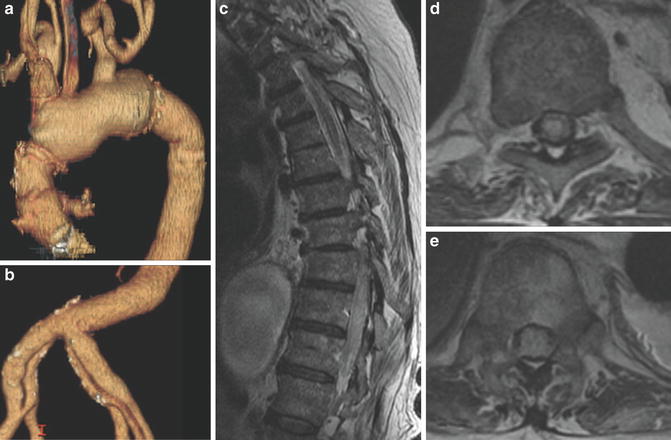
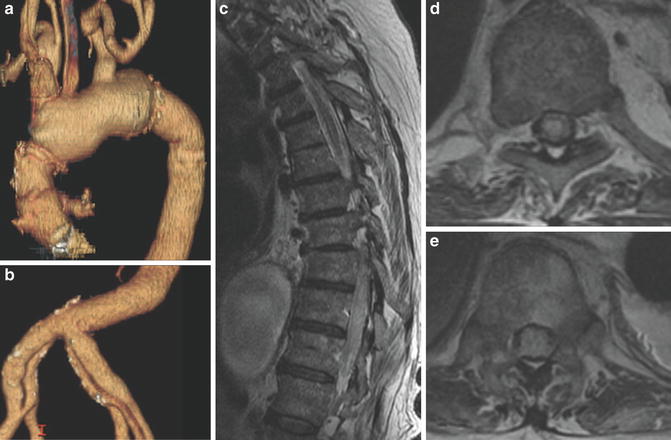
Fig. 4
Spinal cord infarction following aortic surgery: a 69-year-old male with Marfan’s syndrome, status post-graft repair of the ascending aorta (a) following type A dissection, presented with paraplegia following surgery. Note the bilateral iliac artery dissections. (b) MRI – T2-WI hyperintensity in the central gray matter T7/T8 level (c, d) and extends distally to the conus (inferiorly involves both the central and peripheral aspects of the entire distal cord). SCI in the anterior and posterior spinal arteries with cord swelling noted inferiorly (c, e)
Variable incidence of the SCI after endovascular stent graft has been reported up to 10 % [38], but it could be as low as 0.6 % [39] and as high as 28 % in high-risk cases [40]. In a multicenter comparison trial, the reported incidence of SCI after endovascular repair of descending aortic aneurysms has a risk of 3 % versus 14 % for open surgery [41]. SCI incidence is much lower for endovascular repair of the abdominal, rather than thoracic aorta [42]. In the setting of trauma and open repair, the rate of spinal cord ischemia is about 7 % when cross-clamping is used alone. This can be decreased to 3 % by using shunts for distal perfusion and cardiopulmonary bypass [37].
In a large case series, the incidence of paraplegia as a result of thoracoabdominal aneurysm repair was reported as high as 21 %, whereas the aneurysmal repair confined to only the abdominal aorta was 1 % [43]. Spinal cord ischemia has been reported with iliac aneurysm repair as well [44]. Hypoperfusion in the abdominal aorta with prolonged clamping [45], declamping syndrome after declamping of the infrarenal aorta and spinal cord tamponade [46], and increased pressure of the cerebrospinal fluid (CSF) during clamping [47] are the main risk factors in the pathogenesis of the spinal cord ischemia following the aortic surgery.
Spinal cord ischemia may also happen in procedures involving the ligation of radicular arteries, such as spinal tumor resection, scoliosis correction, or pneumonectomy [48].
Atherosclerosis
The link between atherosclerosis and spinal cord ischemia has been considered since the late nineteenth century [49]. Atherosclerosis is minimal in the spinal arterial network when compared to the rest of the body. The anterior spinal artery is the most affected, while other arteries in this system may undergo fibrosis and thickening instead. There is both ascending and descending flows within both the anterior and posterior spinal arterial systems. This allows for collateral circulation in the event of occlusion [50]. While moderate to severe aortic atherosclerosis was observed in 86 % in an autopsy series of SCIs, no meaningful correlation has been observed between degree of aortic atherosclerosis, hypertension, or coronary artery disease and occurrence of lumbosacral ischemia [51]. In a case series of 1,000 autopsies, 28 patients who had atherosclerotic emboli from the abdominal aorta to visceral organs were identified, and the spinal cord was dissected. In 12 cases atheromatous emboli were evident in the spinal arteries, most commonly in the anterior spinal artery. However, only one had a spinal cord infarction, showing that apparently good collateral circulation and lack of general atherosclerosis prevents infarction, unless hypoperfusion leads to decompensation [52].
Aortic Dissection
Spinal cord ischemia as a possible cause of weakness in the limbs as a result of impaired perfusion of the spinal cord aortic dissection had been known since at least the late nineteenth century [53]. Dissecting thoracoabdominal aortic aneurysms, especially those with a left-sided false lumen, are associated with a higher incidence of spinal cord ischemia, because in 85 % of the population, the anterior spinal artery arises from the left lower intercostal vessels [46]. Based on the Stanford classification of the aortic dissection:
Stanford type A aortic dissection – dissection involving the ascending aorta, regardless of the site of primary intimal tear [54] (Fig. 5). Neurological symptoms can be present in 29 % upon presentation. Spinal cord ischemia can be present preoperatively in about 1–9 %. In a study showing a preoperative incidence rate of 1 %, this rate increased to 4 % postoperatively [55].
Fig. 5
SCI following aortic dissection: a 71-year-old male: status post-dissection of the ascending aorta (a, b). Subacute spinal cord infarction involving the central gray matter extends from T11 to the conus medullaris. In addition to the T2-WI hyperintensity (c, d), notice the post-contrast enhancement in the area of infarction (e, f) suggestive of subacute nature (>5 days) of this infarction
Stanford type B aortic dissection – consists of all aortic dissections except type A. Spinal cord ischemia is present in about 3 % of this type [56].
Systemic Hypotension
Based on the autopsy series of 145 fatal cases of cardiac arrest or severe systemic hypotension, SCI was documented in 46 % of cases [51]. Although widely understood that the mid-thoracic is the watershed area, and presumably most susceptible to ischemic injury, the thoracic area was spared, and the lumbar area was the most commonly involved area in 95 % of cases (Fig. 6). Ischemic damage limited to only the thoracic region was seen in only 7.6 % of cases and never in isolation, whereas lumbosacral involvement was the most common affected area, with nearly 70 % of cases of spinal cord ischemia showing only involvement of this region. This is potentially due to higher metabolic demand and a large number of neurons in the lumbosacral region [51]. Similar predisposition of lumbar spinal cord for SCI is also documented in the neonates [57].
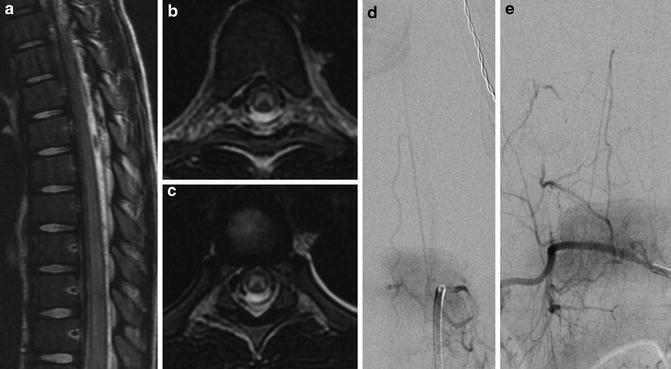
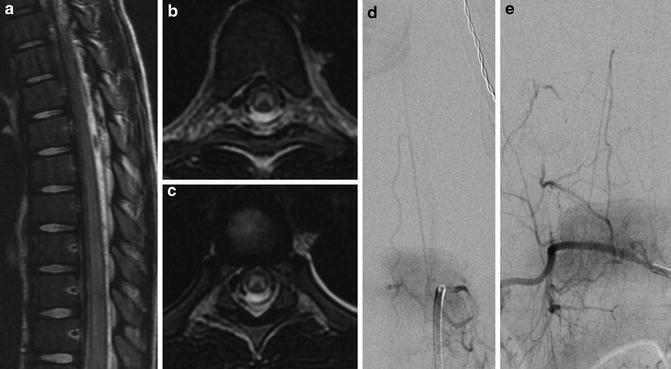
Fig. 6
Anterior spinal artery territory infarct: a 6-year-old girl with gastroenteritis presented with sudden onset of lower extremity weakness, thoracic pain, loss of rectal tone, and mid-thoracic sensory loss. MRI shows diffuse T2 hyperintense signal in the anterior spinal artery territory from T6 to the conus (a–c) in anterior spinal artery syndrome. Subsequent DSA shows patency of AKA (d) and PSA (e)
Surfer’s Myelopathy
This is a rare acute nontraumatic myelopathy, affecting first time/inexperienced surfers. Thompson et al. first described this entity in 2004 [58]. The exact etiology is unknown. Prolonged spinal hyperextension while in prone posture and repetitive flexion and extension are the main precipitating factors resulting in spinal cord arterial insufficiency and watershed ischemia [58]. Proposed vascular mechanisms of injury include avulsion of perforating vessels, vasospasm of the artery of Adamkiewicz, and transient ischemia in areas of borderline perfusion as a result of tension on the spinal cord with hyperextension [59].
Other possible mechanisms include inferior vena cava obstruction and fibrocartilaginous embolism. The former may occur secondary to compression by the liver while lying prone over the surfboard aggravated by concomitant prolonged Valsalva maneuver during paddling, resulting in increased retrograde venous pressure in the epidural venous plexuses leading to infarction [59]. Potential risk factors that may predispose surfers to ischemic cord injury include body habitus (thin with underdeveloped musculature), dehydration (related to travel and prolonged hours at the beach), and long-distance travel (associated with hypercoagulable state and deep venous thrombosis) [58].
The thoracic spinal cord is most commonly affected in the surfer’s myelopathy [60]. The variable levels of thoracic spinal cord involvement may be explained by the variable origins of the artery of Adamkiewicz, arising from T5–T8 in 15 %, T9–T12 in 75 %, and L1–L2 in 10 % of the population [60]. The outcome is variable and does not correlate with the extent or intensity of the signal abnormality on the MRI [61]. Most patients had a complete or near-complete recovery; residual complete paraplegia has been reported [58, 59, 61].
Embolism Related
Thromboembolic Infarction
The main embolic source for the SCI is cardiac, well described in the literature with diverse pathology such as atrial fibrillation, atrial myxoma [62], mitral valve disease [31], and patent foramen ovale [63]. Spinal angiogram may demonstrate the abrupt termination of the anterior or posterior spinal arteries (Fig. 7).
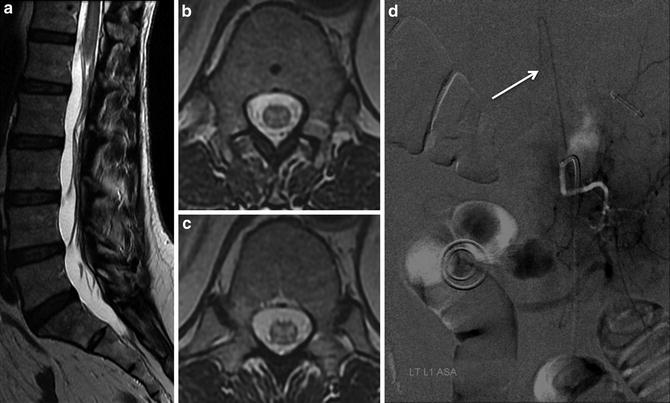
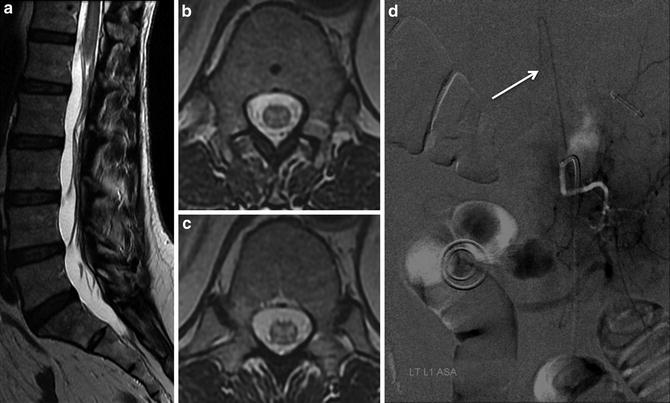
Fig. 7
Embolic spinal cord infarction: a 66-year-old female presented to ED with acute onset bilateral lower extremity motor and sensory deficits. MRI: T2-WI hyperintensity extends from T11 to the conus medullaris involving the central gray matter with cord swelling (a–c). Note the abrupt termination of the AKA (arrow) on subsequent DSA suggesting an embolic occlusion (d) (Courtesy Dr. Kim Nelson and Tibor Becske, NYU Medical Center, New York, NY)
Fibrocartilaginous Embolism
Fibrocartilaginous embolism (FCE) was first described as a cause of spinal cord infarction in 1961 [64]. A history of heavy lifting or trauma to the spine may be a clue to this etiology, but this may not always be the case. Nearly 70 % of cases are young women, in contrast to thrombotic or other embolic etiology, predominantly affecting men. Three characteristics distinguish FCE from other thrombotic or embolic causes of SCI [65]. First, their presenting symptom is that of the sudden severe pain in the region of the spine that is affected, whereas in thrombotic and other embolic causes, this is hardly ever the case. Second, after the onset of pain, there is a period with no neurological symptoms. Over the course of 15 min to 48 h, symptoms of neurological deficit progress, indicating the evolution of a spinal stroke, whereas in thrombotic and other embolic etiologies, numbness and weakness of the legs begin at onset and at the same time as pain, if present. Finally, these cases have a very poor outcome, while those of thrombotic or other embolic causes often improve over time.
It is thought to occur when, due to increased intra-disk pressure, fragments of the intervertebral disk are pushed into the disk vasculature or the bone marrow and venous sinuses of vertebral bodies. Supporting this is the finding of fibrocartilaginous emboli in the bone marrow and vascular supply of the affected regions in autopsies [65, 66]. Seventy percent involve the upper cervical cord, with some extending to the lower medulla oblongata or the lower thoracic, which explains the high fatality of these cases. The presence of cord swelling and collapsed intervertebral disk at the corresponding level indicates this etiology [65].
Infection
Syphilis was diagnosed in a majority of cases of spinal cord ischemia in a report from 1902 [5]. This was prior to the introduction of penicillin as a cure. Later case series show the most common identified etiology to be of aortic origin, with many as a complication of surgery on the aorta [9]. Bacterial meningitis [67] and mucormycosis [68] are reported in the literature as an etiology for SCI.
Iatrogenic Embolization
The spinal cord ischemia has been reported as a complication related to therapeutic embolization. This is particularly reported with bronchia artery embolization for hemoptysis between 1.4 % and 6.5 % [69]. Many other iatrogenic procedures are reported to cause spinal cord infarction including but not limited to renal artery embolization [70], intercostal embolization in hemoptysis [71], intercostal artery embolization in traumatic hemorrhage [72], endoscopic variceal sclerotherapy [73], ligation [74], regional anesthesia, and pain injections [75].
Spinal Angiography and Embolization
Angiography of the thoracic or abdominal branches of the aorta rarely results in spinal cord ischemia. Spinal angiography is more challenging in patients with atherosclerotic aorta and thoracoabdominal aneurysm. Spinal cord infarction as a complication of angiography was documented as early as 1949 and reported on in the decade thereafter [76]. However, with increased experience and better techniques and equipment in this field, complications have been lowered. In a series of 134 consecutive spine angiograms in 63 patients published in 1988, three neurological complications were reported (incidence 2.2 %), all of which resolved in a matter of 1 day to 1 week [76]. In more recent series on spinal angiography and embolization from high-volume centers [77] including authors’ experience with 220 spinal angiograms and 180 embolizations [78], no neurological complications were reported.
Hemorrhagic Spinal Cord Infarction
Spinal subarachnoid hemorrhage (SAH) accounts for 1 % of all SAH. However, this is the most common form of hemorrhagic spinal cord infarction (Fig. 8). Cervical spinal cord ischemia has been described in association with spinal subarachnoid hemorrhage in patients following dissection of the vertebrobasilar system or ruptured vascular malformations of the spinal cord [77]. Disruption of the blood supply due to the involvement of radiculomedullary arteries is thought to be the underlying mechanism in the acute setting [78]. Micro- and macrocirculatory failure secondary to subarachnoid hemorrhage may result in delayed ischemic deficits. Vasospasm has shown to be the most likely mechanism causing secondary injury in experimental animal models of spinal cord injury [79].
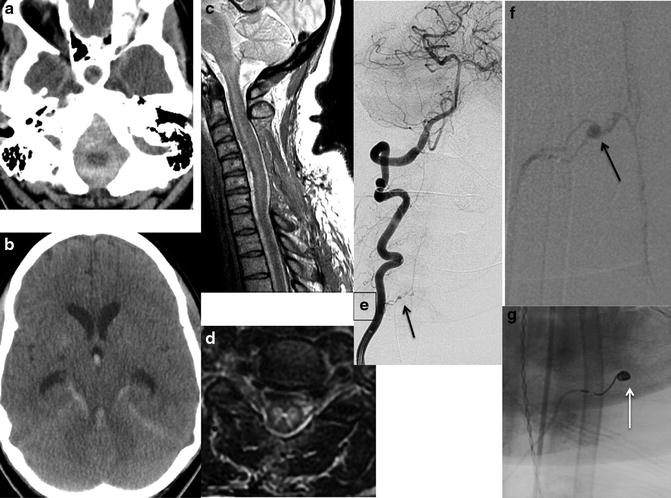
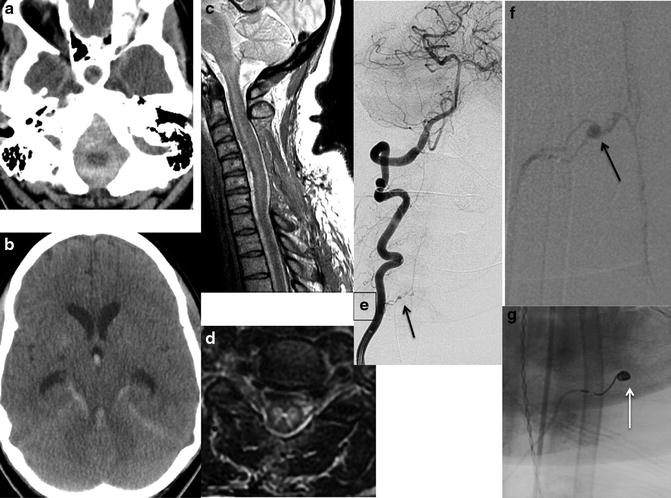
Fig. 8
Spinal cord infarction from SAH: a 54-year-old female presented with acute subarachnoid hemorrhage (SAH) and quadriplegia. CT – posterior fossa predominant SAH with intraventricular extension and hydrocephalus (a, b). MRI – posterior decompression of the cervical spine with residual diffuse T2-WI hyperintense cord signal involving the central gray matter with edema and cord swelling (c, d). Also notice the tracking of the extra-axial hemorrhage from posterior fossa along the anterior border of the spinal cord with dorsal displacement (c). DSA demonstrates a small anterior spinal artery aneurysm (arrow), as a culprit of SAH, pre- (e, f) and post- (g) successful coil embolization projections
Although different lesions predispose to hemorrhage in different compartments (Table 2), their strict localization is not possible. The hemorrhage tends to extend across multiple compartments depending on the amount and site of hemorrhage and may result in subdural and epidural hematoma in addition to the subarachnoid hemorrhage. Prolonged mass effect from the extra-axial hematoma in addition to vascular disruption related to involvement of the radiculomedullary feeders, circulatory failure, and vasospasm might result in spinal cord ischemia in these scenarios [78]. Conditions such as anticoagulation and bleeding diathesis may cause hemorrhage in either compartments.
Spinal Venous Infarction
This less common etiology is usually associated with spinal dural arteriovenous malformations (Fig. 9) secondary to venous congestion [80] and is discussed in multiple subsequent sections of this chapter. It may also be a complication of deep sea diving, in which gaseous occlusion of the spinal venous plexus has been postulated as a mechanism of injury in spinal decompression myelopathy [9]. Early recompression reverses symptoms in about three-fourths of patients [81, 82]. Kim et al. [83] stated that the hemorrhagic, nonhemorrhagic, and embolic venous infarction could not be differentiated with certainty on clinical grounds. Hemorrhagic venous infarctions were associated with marked pain at the time of onset and progressed rapidly; survival time was relatively short. Nonhemorrhagic infarctions were less often painful and evolved slowly. Survival time was longer than in hemorrhagic infarction. Embolic venous infarction was associated with pain of abrupt onset. As the initial presentation does not correlate with severity of the disease, treatment with hyperbaric chamber should be initiated promptly in decompression sickness, even in those with mild symptoms [82]. Also, the potential association of malignancy and venous spinal cord infarction is well recognized, particularly in the case of pancreatic neoplasm [30].
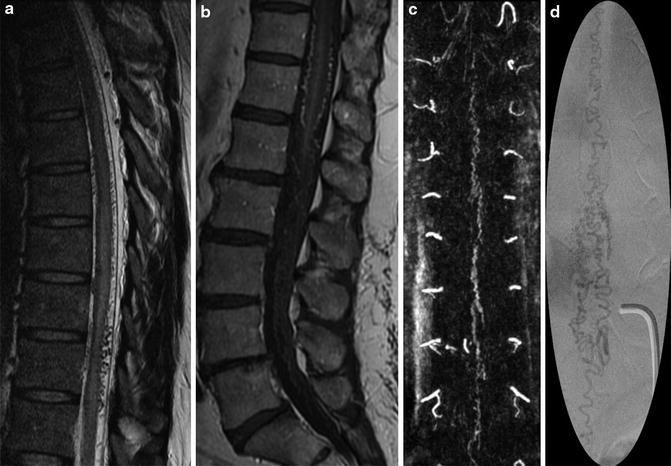
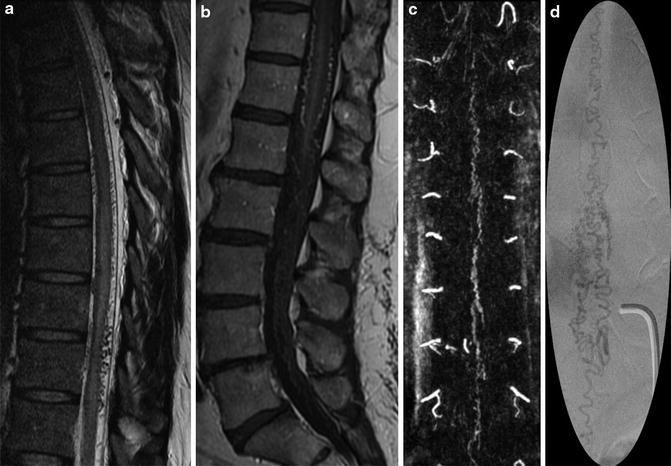
Fig. 9
Spinal dural arteriovenous fistula (DAVF): a 42-year-old male with progressive paraplegia. MRI – diffuse serpiginous flow voids over the cord surface with associated T2-WI hyperintense cord edema and swelling (a). Contrast administration reinforces the visualization of blood vessels over cord surface (b). Reconstructions from the contrast-enhanced time-resolved MRA demonstrate the spinal DAVF (c). This is confirmed on the subsequent spinal DSA (d), supplied by the right T10 radiculomedullary artery
Pathophysiology
Understanding of the biomolecular changes that occur during and after spinal cord infarction (SCI) is essential for all those involved in the care of these patients. Extensive research over the past 30–40 years has focused on elucidating acute spinal cord injury including both primary and secondary mechanisms, slowly unraveling the complex pathophysiologic processes such as roles of calcium, free radicals, sodium, excitatory amino acids, vascular mediators, and apoptosis. These concepts of primary and secondary mechanisms and their duality in acute spinal cord injury have since also been embraced in the understanding of the pathophysiology of spinal and cerebral ischemia, subarachnoid hemorrhage, and head trauma [84].
Concepts of Primary and Secondary injury
Fehlings proposed a two-step process involving primary and secondary mechanisms in acute spinal cord injury [85]. Allen first postulated the concept of a secondary mechanism in 1911 based on canine studies [86, 87].
Primary Mechanism
The primary mechanism involves the initial precipitating event such as atherosclerosis, cardiac arrest, thromboembolism, hypovolemic shock, and hemorrhage with associated compression. Similar to the brain, spinal cord vessels autoregulate according to SBP and PaCO2, but the spinal cord perfusion is more directly affected by changes in systemic blood pressure. The “watershed region” of the spinal cord in the mid-thoracic region (T4–T8) is anatomically more susceptible for the ischemic injury. In case of spinal arteriovenous malformations, the shunting of the arterial blood away from the spinal cord (arterial steal phenomenon) or venous hypertension may result in hypoperfusion. The hypoxia and hypoperfusion resulting from the primary mechanisms trigger the secondary mechanism.
Secondary Mechanisms
Over the past 40 years, various theories of secondary mechanisms of SCI have undergone a process of maturation. In the 1970s, Demopoulos et al. [88] proposed the free radical hypothesis, as crucial to the injury process. Ten years later the focus shifted onto the role of calcium, opiate receptors, and lipid peroxidation. Research in the twenty-first century has suggested apoptosis, intracellular protein synthesis inhibition, and glutaminergic mechanisms, among a myriad of pathophysiologic pathways that mediate secondary injury mechanisms. There is considerable evidence that the primary mechanical injury initiates a cascade of secondary injury mechanisms [85, 89–93].
Although there are many mediators of secondary injury, vascular mechanisms, free radicals, and more recently apoptosis have received much attention.
Free Radicals
Free radicals are highly reactive molecules that possess an extra electron in the outer orbit. Free radicals most commonly form from molecular oxygen. There is good evidence for the early occurrence and pathophysiologic importance of oxygen free radical formation with cell membrane lipid peroxidation in central nervous system injury [88]. Severe uncontrolled production of the hydroxyl radicals (HO) from dissociation of hydrogen peroxide (H2O2) can cause lipid peroxidation, impairment of phospholipid-dependent enzymes, disruption of ionic gradients, and ultimately the lysis of cell membrane [94, 95]. Lipid peroxidation may also play a role in postictal hypoperfusion after initial precipitating event and may result in SCI [94].
Vascular Mechanisms
The changes that occur in spinal cord blood flow after an acute spinal cord injury can be divided into systemic and local.
Local effects: Following acute cord injury, a major reduction in blood flow occurs at the site of ictus, i.e., embolus or hemorrhagic compression, possibly precipitated by vasospasm or vasoactive amines [96, 97]. Based on animal studies, autoregulation is intact during the initial 60–90 min after cord injury but is then lost coincident with the onset of ischemia. It has been suggested that the ischemic response to cord injury is mediated both by the loss of autoregulation and by relative constriction of the resistance vessels [98]. Ischemia may play a role in the formation of local cord edema, although whether edema formation is injurious in itself or an epiphenomenon is still unclear [84].
Because of differences in relative vascularity, the central gray matter, along with adjacent white matter, is more severely affected by acute cord injury than peripheral white matter [99]. Primate studies have shown that the gray matter has higher blood supply than the white matter due to the much higher metabolic rate. Also, the flow was more variable with lower values in the dorsal horns and higher values in the central gray and anterior horns, compared to the more uniform flow in the white matter [100]. The blood flow to the corticospinal tracts was considerably higher than the rest of the white matter, suggestive of higher metabolic activity in these tracts and increased predisposition to the ischemia [101].
The intrinsic arterial blood supply to the spinal cord is directly proportional to the cross-sectional area of the gray matter, which is most abundant in the thoracolumbar segment [102]. Therefore, in normal conditions, the blood flow to the spinal cord is highest in the thoracolumbar segment, which has the highest concentration of central arteries owing to the relative abundance of gray matter. Consequently, the same segment is the most vulnerable to hypoperfusion, particularly the gray matter that corresponds to the lumbar enlargement of the spinal cord. Arterial infarcts of the posterior spinal artery territory are rare compared with the anterior spinal artery territory. The scarcity of this condition is most likely due to extensive anastomosis of the posterior spinal artery [103] and relative resistance of the posterior column to the ischemia due to its inherent lowest blood flow of all the tracts in the spinal cord [101].
White matter perfusion typically decreases within 5 min of an acute cord injury and begins to return to normal within 15 min. It remains thereafter near-normal pre-injury values during the first 24 h. In contradistinction, in the central gray matter, numerous microhemorrhages typically occur, as early as 5 min after an acute cord injury. Perfusion is relatively absent 1 h after an injury, and this state remains so for at least the first 24 h. This vascular standstill has been confirmed using microangiography [97, 104], fluorescent tracer studies [105], and the operating microscope [106] and plays a major role in the devastating outcomes from acute cord injury. Alterations in endothelial cell function causing an increase in vascular permeability and edema formation have been well documented [107–109].
Systemic effects: After an acute SCI, an immediate but transient increase in mean arterial blood pressure may occur followed by profound hypotension and bradycardia [110]. The reason for this transient hypertension is unknown but may be mediated by both the thoracic sympathetic ganglions and the adrenal glands [111]. Acute cord injury is one of the causes of neurogenic shock [112], typically being related to the magnitude and severity of the cord injury.
Of all purported mechanisms of secondary injury, the vascular hypothesis has considerable weight, with biochemical, angiographic, histopathologic, and clinical support for its key role in damage after acute SCI.
Apoptosis
Apoptosis also called programmed cell death occurs in a wide variety of disease states in eukaryotic cells. Apoptosis is recognized as occurring in utero as a form of neuronal cell death during embryonic development [113] and is also now thought to play a role in many post-developmental disorders of the central nervous system, including ischemia [114]. Apoptosis is an active process that is characterized by cell shrinkage, chromatin aggregation, and nuclear pyknosis [115]. In the spinal cord, apoptosis was first identified in 1995 as occurring in rats [116] and, more recently, in the human spinal cord [117]. This is a tightly regulated process with a sequence of activation steps that require energy and specific macromolecular synthesis as de novo gene transcription [115]. A family of cysteine proteins, the caspases, is thought to play an important role in apoptosis, especially caspase-3 [84]. Oligodendrocytes are the major cell type in compressive cord injury that undergo apoptosis [118], seen in areas of wallerian degeneration and detectable between 24 h and 3 weeks post injury [116].
Spinal Venous Infarction
Spinal cord veins are valveless. However, an anti-reflux mechanism has been described at the dural exit of radicular veins. It consists of an increased smooth muscle fibers and a Z-shaped course of the radicular vein when it crosses the dura to drain spinal cord venous blood in the epidural veins [14]. In case of spinal dural arteriovenous fistulas, the arterialized vein drains the fistula blood flow toward the spinal cord, and the increased pressure is transmitted to the intrinsic cord veins in retrograde fashion. This results in decreased intramedullary arteriovenous gradient and hypoxia, loss of autoregulation, and disruption of blood-cord barrier and, if left untreated, results in subacute necrotic myelopathy.
Imaging Correlation
Ischemia in the distribution of the anterior spinal artery rapidly initiates vasogenic and cytotoxic edema in the gray matter with subsequent increase in mobile water. This is manifested by a focal increase in proton density and a prolongation of the T2 relaxation time, which appears as distinct increase of signal intensity on the long TR pulse sequences.
The ischemia/signal abnormalities start in the anterior horns of the gray matter and, with increasing severity, spread posteriorly to involve the posterior horns. Ultimately, the ischemic changes and corresponding MR signal abnormalities extend laterally to the posterolateral funiculi of the spinal cord, including the crossed corticospinal tracts. In severe cases, the whole cross section of the spinal cord is infarcted. There seems to be a correlation between the distribution of the MR signal abnormalities and the severity of the clinical symptomatology seen in spinal cord ischemia [46] (Table 3).
Table 3
Summary of secondary injury in acute spinal cord infarction
Ischemia |
Impaired autoregulation |
Neurogenic shock, hemorrhage |
Microcirculatory derangements |
Vasospasm |
Thrombosis |
Increased intracellular calcium |
Increased extracellular potassium |
Increased sodium permeability |
Neurotransmitter accumulation |
Catecholamines [121] (e.g., norepinephrine, dopamine) |
Arachidonic acid release [124] |
Edema [129] |
Decreased adenosine triphosphate production [130] |
Clinical Presentation
Acute spinal cord infarction syndrome (ASCIS) can result in various clinical manifestations based on the vasculature affected and etiology, but has common symptoms. Bilateral weakness, sensory loss, back pain at onset, and urinary retention were the most common symptoms at the time of presentation in a prospectively collected series of 28 patients [134]. The most commonly affected location is the thoracolumbar level, and paraparesis comprises of 20–25 % of all spinal cord infarction cases [135]. Pain and sensory changes occur first, followed by weakness within minutes to hours, which peaks in 12 h [135]. Almost invariably spinal cord infarction presents with pain, at the level of the lesion, one retrospective study noting pain in 72.7 % of 23 patients [136]. Transient ischemic attacks (TIA) rarely precede spinal cord infarct, comprising of 10 % of spinal cord infarcts [135]. TIA manifest as weakness of the lower limbs precipitated by physical activity or as “drop attacks” for cervical cord ischemia.
Ischemic Spinal Cord Infarction
Based on international standards for neurological and functional classification of spinal cord injury proposed by the American Spinal Injury Association (ASIA) and International Medical Society of Paraplegia (IMSOP), ASCIS can be categorized into four groups [137]. The clinical syndromes are:
1.
Anterior spinal artery syndrome (ASAS)
2.
Posterior spinal artery syndrome (PSAS)
3.
Brown-Sequard syndrome (BSS)
4.
Complete spinal cord transaction (CSCT)
Anterior Spinal Artery Syndrome (ASAS)
This is the most common presentation of ASCIS, affecting the anterior two-thirds of the spinal cord that is supplied by one anterior spinal artery (Fig. 10). Cervical anterior cord infarction manifests with diffuse or radicular neck pain followed by quadriparesis, associated with anal or bladder sphincter dysfunction and sensory loss of pain, pinprick, and temperature modalities. Proprioception and vibration sensations, which are usually spared in anterior cord syndrome, can be affected in anterior cervical lesion due to involvement of medial lemniscus. Later on, flaccidity and areflexia give way to spasticity and hyperreflexia. Patients present with acute interscapular pain, followed by paraparesis or paraplegia, urinary and rectal incontinence or retention, and a sensory loss to pain and temperature most common at the T4 level. Lumbosacral region is affected by lesion in the radicular artery of Adamkiewicz. Extensive lesions can be seen with flaccid paraplegia and autonomic dysfunction, including respiratory compromise with cervical lesions. Rarely, one segmental branch of anterior spinal cord artery can result in monoparesis or hemiparesis, instead of paraparesis [138].
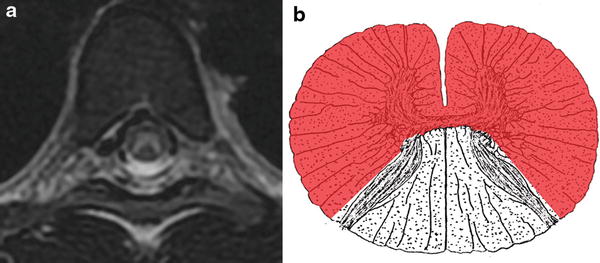
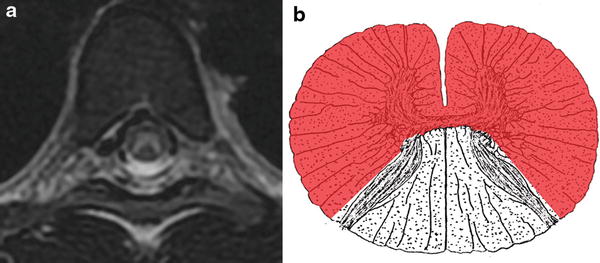
Fig. 10
Anterior spinal artery (ASA) territory infarction. T2-WI hyperintense signal abnormality involving the anterior two-thirds of the spinal cord including both gray matter and white matter secondary to occlusion of the ASA (a). Pictorial representation of the ASA territory infarction (b)
Posterior Spinal Artery Syndrome (PSAS)
Posterior cord infarction is rare due to the rich anastomotic network (Fig. 11). Posterior column and dorsal horns are affected, manifested as total anesthesia and areflexia at the level of lesion. Affected patients have deficit in vibratory and proprioception deficits below the lesion.
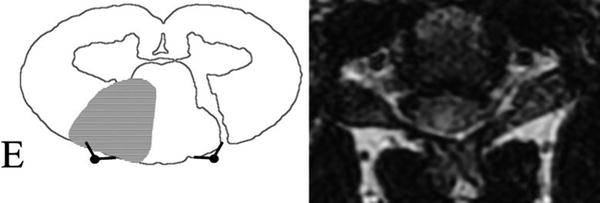
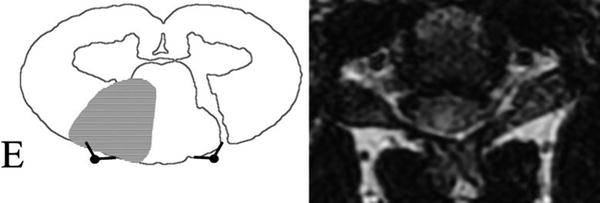
Fig. 11
Posterior spinal artery (PSA) territory infarction. Schematic representation of the posterior cerebral artery (PCA) territory infarction on the right. Note the corresponding MRI on the left with subtle focal T2-WI hyperintensity in the right PSA territory of the spinal cord
Brown-Sequard Syndrome (BSS)
Central sulcal artery occlusion can cause Brown-Sequard syndrome, which typically presents with ipsilateral weakness and posterior column sensory deficit (vibration and proprioception) below the lesion and contralateral decrease in pain and temperature sensation a few segments below the lesion.
Central Spinal Cord Syndrome (CSCS)
Central cord syndrome may theoretically be a manifestation of spinal cord ischemia, but commonly result from cervical spondylosis, trauma, syringomyelia, or other tumors. Location is the cervical or upper thoracic regions, involving the central portions of the spinal cord. The hallmark sign is characterized by weakness in the arms, with relative sparing of the legs, due to the latter’s corresponding corticospinal tract in lateral part of spinal cord. Affected patients also have varying degree of sensory loss below the lesion and urinary retention [139].
Hemorrhagic Spinal Cord Infarction
Hemorrhagic infarction presents with sudden onset of pain, usually at the level of hemorrhage. Meningeal irritation is a typical finding. Patients may have associated headache, cranial neuropathies, and decreased level of consciousness depending on extent and distribution of the hemorrhage. The evaluation for spinal SAH typically follows negative intracranial workup.
Venous Hypertension
This is the most common presentation in SDAVF. Patients present with progressive lower extremity weakness (>50 %), sensory deficits (30 %) including paresthesia, sensory loss and hyperesthesia, gait disturbance, back pain (24 %), bowel and bladder disturbance, and impotence. Once motor or sensory deficits appear, severe disability ensues over 6 months to 2 years.
Imaging Findings
Magnetic resonance imaging (MRI) is the primary imaging modality of choice for the diagnosis of suspected spinal cord infarction due to its superior spatial and contrast resolution for differentiation of the intraspinal soft tissues and detection of intrinsic cord pathology [140] (Fig. 12). Also MRI is most sensitive in the detection of the marrow changes of the adjacent vertebral bodies [140]. Perhaps the most important role of MRI in both ischemic and hemorrhagic SCIs is to rule out alternative diagnosis. MRI not only confirms the evidence of spinal cord infarction but also may provide information as to the underlying etiology. The extent of signal abnormalities correlates well with the severity of the clinical findings and the potential for recovery [46]. Following acute presentation, MRI should be performed on emergency basis to confirm the diagnosis, delineate pattern of SCI, and rule out differential diagnosis such as cord compression from traumatic, degenerative, or neoplastic processes and intramedullary lesions such as acute inflammation, hemorrhage, and congestive ischemia due to vascular malformations.
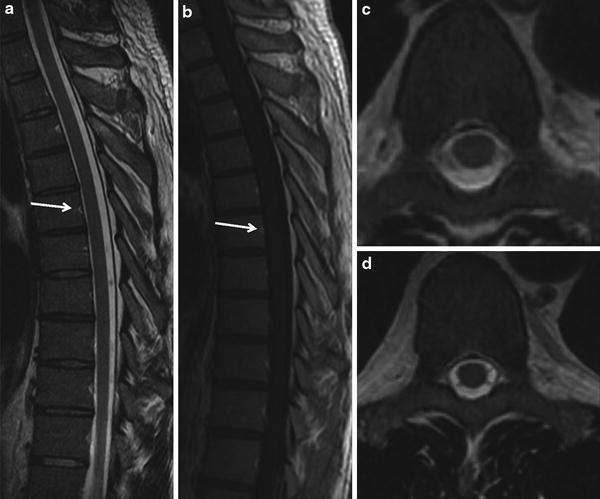
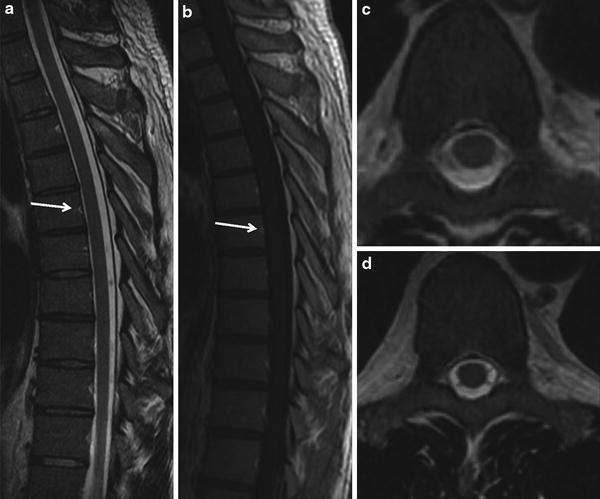
Fig. 12
Normal MR imaging of the spinal cord. Homogeneous cord signal, no intrinsic T2 hyperintensity of the cord or cord swelling or serpiginous flow voids on the cord surface (a, c, d). No vascular enhancement either intrinsically within the cord or vascular enhancement over the cord following contrast administration (b). The focal T2-WI hyperintensity and post-contrast enhancement (arrows) in the middle of the posterior vertebral border represent a vertebral vasculature, a normal finding. Note the exquisite spatial and soft tissue contrast resolution offered by the MRI in spine imaging
Evolution of Spine Imaging
Low signal-to-noise ratio (SNR) and prolonged imaging times while scanning the entire spine are the main challenges of the spine imaging. Introduction of the phased-array coil technology [141] and increased field strength has increased the baseline SNR, a major improvement in spine imaging, which has inherently limited SNR. Similarly introduction of the parallel imaging [142, 143] and improvement of multielement radiofrequency (RF) coils have not only enhanced the SNR of high-resolution MR imaging over a large craniocaudal extent but also dramatically shortened the imaging times.
Standard Spine Protocol
Regular structural MR imaging sequences for diagnosis of the cord infarction include multiplanar T1- and T2-WI fast spin-echo (FSE), gradient-echo (GRE), and contrast-enhanced (CE) acquisitions. Diffusion-weighted imaging (DWI) has increasingly shown as a promising technique in the evaluation of the acute spinal cord syndrome [144, 145] and used as a part of the standard protocol in the evaluation of acute non-compressive myelopathy.
Conventional MRI
The sensitivity of conventional MRI is limited, particularly in the first several hours. The reported sensitivity of T2-WI cord signal abnormality in SCI ranges between 45 % and 73 % depending in part on timing of the scan [1, 9, 10, 146, 147]. Hence the initial scan may either confirm the diagnosis or be a normal study and serve as a baseline for future follow-up imaging. Isolated finding of T2 signal abnormality, even with restricted diffusion, is not specific for ischemia and can be seen in transverse myelitis and other intrinsic cord pathologies. These findings, confined to a vascular territory, are more specific for SCI.
Diffusion-Weighted Imaging (DWI) of the Spinal Cord
Historical Aspects
DWI of the spinal cord is technically challenging, but is currently feasible. Hajnal et al. are the first to publish the DW images of the human cervical spinal cord in 1991 [148]. By using a standard EPI sequence and a spinal coil, Stepper and Lovblad [149] demonstrated areas of hyperintensity on DWI (ischemic region) corresponding to a decrease of the local ADC (75 % of normal values) when compared with non-affected spinal cord. The time course of the changes in the ADC values corresponds to what has been reported in the brain [150].
Technical Challenges
Several technical difficulties for the application of the most frequently available DWI technique, i.e., single-shot echo-planar imaging (EPI), to spinal cord imaging include motion artifacts caused by physiological movement of the spinal cord and surrounding structures (e.g., cerebrospinal fluid pulsatile flow, swallowing, respiration, and cardiac motion), susceptibility artifacts caused by the presence of bone and cerebrospinal fluid interfaces, and the low signal-to-noise ratio caused by the small pixel dimensions required for appropriate visualization of the spinal cord [151]. Improved imaging techniques such as navigated interleaved EPI or parallel imaging-enhanced EPI provide superior resolution and adequate resistance to motion artifacts compared to the conventional EPI [140]. More recently, line scan diffusion imaging (LSDI) has been reported as new advancement in the DWI of the spinal cord [151].
Clinical Role
Increasingly, DWI has shown to be highly sensitive to the early detection of ischemic lesions in spinal cord and thus can improve the diagnostic capabilities especially in the acute stage [152–154]. DWI is also a very useful technique in differentiating diagnosis of subacute infarction. After all, hyperintensities on T2-WI images are not specific for ischemia and can also be seen in inflammatory diseases and tumors [155]. DWI will have increasingly important role for ischemic diseases of the spinal cord not only in early diagnosis but also in differentiating diagnosis [155].
Typical MRI Findings
The typical MRI findings in spinal cord infarction include isolated pencillike area of T2 hyperintensity involving the centromedullary region with sparing of the anterior rim, often encompassing more than two vertebral segments on sagittal images [2, 156], and bilateral hyperintensities that are mostly confined to the anterior horn area, leading to the typical “snake eyes or owl’s eyes” configuration on the axial acquisitions [156, 157]. Although the T2 hyperintensities are seen in 90 % of patients with SCI, these are nonspecific [156]. Further in the acute phase, only 50 % of the patients show a demarcation of T2 hyperintensity of the spine during the first 24 h which limits the sensitivity of this finding [80].
A finding of restricted diffusion on diffusion-weighted images (DWI-MRI) reported to be significantly more sensitive than standard T2 images, appearing as early as 3–8 h after the ictus, but the limited evidence precludes a numerical estimate of its sensitivity [144, 151, 152, 158]. Studies have shown that restricted diffusion can be seen in SCI as early as 3–8 h following an ictus [152–154]. Moderate swelling of the cord is noted in the acute phase with onset of T1-WI hypointensity in the cord from days 5–7 [80]. Contrast enhancement is noted in the subacute stage, typically after 5 days, and persists for up to 3 weeks after onset [159]. Similar to the cerebral edema, venous hypertension from spinal DAVF without cord infarction gives pure extracellular edema, thus increasing T2-WI signal intensity but with normal findings on DWI.
Stages of Spinal Cord Infarction
The abnormal signal intensities in SCI typically start in the territory of the anterior spinal artery and spread to the adjacent central white matter. The patterns of MR signal abnormality with increasing signal changes reflect a temporal sequence of ischemic changes that can often be recognized as distinct, separate stages. However, the MR appearance is frequently a mixture of patterns, particularly in the patients with completed spinal cord stroke.
Depending on the extent of involvement on MRI, spinal cord ischemia can be categorized into four patterns [46]:
Anterior Horn Ischemia
Results in abnormal T2-WI hyperintense signal involving the anterior horns of the gray matter giving an “owl’s eye” appearance (Fig. 13a).
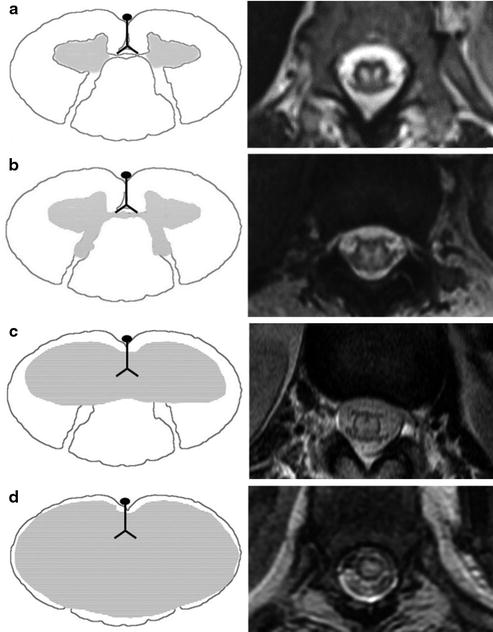
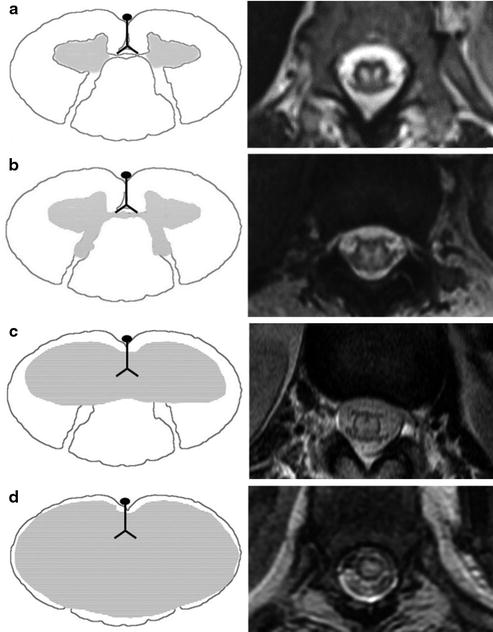
Fig. 13
(a) Anterior horn infarction. Schematic representation of the anterior horn infarction on the right. Note the corresponding MRI on the left with subtle focal T2-WI hyperintensity in the anterior horns of the spinal cord. (b) Central gray matter infarction. Schematic representation of the central gray matter infarction on the right. Note the corresponding MRI on the left demonstrating focal T2-WI hyperintensity in the central gray matter of the spinal cord. (c) Central gray and white matter infarction. Schematic representation of the central gray and white matter infarction on the right. Note the corresponding MRI on the left depicting the diffuse T2-WI hyperintensity involving the central gray and white matter of the spinal cord. (d) Cross-sectional infarction. Schematic representation of the cross-sectional infarction on the right. Note the corresponding MRI on the left with diffuse focal T2-WI hyperintensity involving the cross-sectional area of the spinal cord. Note the thin rim of preserved normal cord parenchyma secondary to the perfusion from peripheral pial plexus
Central Gray Matter Ischemia
T2-WI hyperintense signal abnormality involving both anterior and posterior horns of the gray matter (Fig. 13b).
Gray and White Matter Ischemia
T2-WI hyperintense signal abnormality involving the entire gray matter and the adjacent white matter (Fig. 13c).
Cross Section Ischemia
T2-WI hyperintense signal abnormality involving the entire cross section of the spinal cord involving both gray matter and white matter (Fig. 13d).
Associated Vertebral Body Infarction
A finding of vertebral body infarction on MRI is a useful indicator of vascular nature of the acute spinal cord syndrome [160–163]. However, this finding is not specific, seen in only 4–35 % of patients with spinal cord infarction [1, 146, 164]. SCI may not always be accompanied by vertebral body infarction, because arterial occlusion may be located distal to those vessels supplying the vertebral body or because of the good collateral blood supply of the vertebra [160]. Cheng et al. [164] showed that concomitant spinal cord and vertebral body infarction is highly associated with aortic pathology, which highlights the common blood supply segmental arteries supplying the metamere and radiculomedullary branches of the radicular artery (branch of spinal trunk of the segmental artery) feeding the spinal cord [13].
Useful Clues on MRI to the Underlying Etiology
Holistic Approach
In a patient with suspected SCI, the MRI signal abnormalities alone are nonspecific. Care should be taken to interpret these findings in conjunction with history, clinical findings, and CSF analysis. Although CSF analysis in spinal cord infarction is typically normal, but there can be pleocytosis (rarely more than 100 WBC) and an elevated protein (usually less than 119 mg/dl) [7, 9, 10, 166].
Advanced Techniques
Advanced techniques such as diffusion tensor imaging (DTI) and fiber tractography (FT) are increasingly investigated in the cord infarction. These techniques have technical challenges limiting the image quality, related to the highly magnetically inhomogeneous material surrounding the spinal canal, small size of the spinal structures, long craniocaudal extent of the spinal canal, pulsation effects of cerebrospinal fluid (CSF) and blood, and motion degradation from respiration, swallowing, and patient movement. In patients with cervical cord injury, fractional anisotropy (FA) of DTI and FA showed better correlation with motor function and clinical findings such as neurological impairments compared to the conventional MRI [167]. DTI imaging of the spinal cord is currently only a research tool, but preliminary studies have shown that it holds considerable promise in predicting the severity of spinal cord injury [168].
Follow-Up Imaging
Unlike cerebral infarct, onset of T2 hyperintense signal abnormality in the spinal cord infarction is variable from 8 to 72 h, and studies reported normal MRI findings at 48 h following ictus [157, 169]. High index of clinical suspicion warrants follow-up imaging. However, up to 14 % of patients with suspected SCI have normal follow-up MRI scans [10, 166].
Repeated or follow-up DWI may also help in differentiating diagnosis. There seems to be more sensibility on DW images than on T2-WI images during signal changes within the course of disease, and DWI may be useful in monitoring the progress of the disease [155]. Serial MR studies with contrast showed sequential changes of enhancement similar to those seen in cerebral infarcts [169]. Understanding of characteristic MR findings and evolutional changes at different stages of SCIs and follow-up using serial MRI with contrast are vital in the early diagnosis and more effective in the management of the patients.
Differential Diagnosis
The differential diagnosis of spinal cord infarction is broad and holds multiple entities under the roof of “acute nontraumatic myelopathy.” These can be categorized into (a) inflammation, (b) infection, (c) compressive myelopathy, (d) venous congestion related to vascular malformations such as spinal dural arteriovenous fistula (SDAVF), and (e) tumor. Distinction of SCI from “SCI mimics” is challenging especially in subacute stage. After all, hyperintensities on T2-WI images are not specific for ischemia and can also be seen in inflammatory diseases and tumors [155].
Sudden onset of symptoms may support the diagnosis of medullary ischemia. In addition, due to the characteristics of blood supply in the spinal cord, infarction lesions are confined to arterial territories and usually lie in the anterior or posterior part of the spinal cord, whereas inflammatory or neoplastic medullary lesions often occupy or affect the whole medulla. Detection of synchronous lesions in the brain may isolate some of the differential diagnosis (Table 1).
DW MRI will have increasingly important role for ischemic diseases of the spinal cord not only in early diagnosis but also in differentiating diagnosis [155]. Marked hyperintensity on DWI may provide another indicator leading to the specific diagnosis of spinal medullary ischemia. Mild hyperintensity was likely to be seen on DW images of myelitis or tumors, suggesting the possibility of separating ischemic from inflammatory cord lesions and intramedullary tumors by the different range of ADC values [153, 155]. Repeated or follow-up DWI may also help in differentiating diagnosis. There seems to be more sensibility on DW images than on T2-WI images during signal changes within the course of disease, and DWI may be useful in monitoring the progress of the disease [155].
The list of differential diagnosis is summarized in the table. Main differential diagnoses are discussed below.
Multiple Sclerosis (MS)
Multiple sclerosis is a chronic inflammatory demyelinating disease of the central nervous system (CNS). The frequent involvement of spinal cord in MS is shown up to 99 % in autopsy series [170]. 70–80 % of the patients with MS have T2-WI cord signal abnormalities [171]. MS plaques are best demonstrated on the T2-WI acquisitions, with signal characteristics of T2-WI hyperintensity and T1-WI iso-hypointensity [172], similar to cord infarction. Cord swelling is a usual feature of the relapsing-remitting form of MS [173, 174], and similarly cord atrophy with axonal loss is a recognized form of MS [175]. Post-contrast enhancement of the demyelination lesions is described due to the associated breakdown of the blood-brain barrier, although significantly lower than their brain counterpart [176].
However, the MS lesions differ from SCI in pattern of cord involvement and extent of CNS distribution. MS plaques present as well-circumscribed foci of T2-WI hyperintensity with asymmetric involvement of the cord. They are characteristically peripherally located, are less than two vertebral segments in length, and occupy less than half the cross-sectional area of the cord. On sagittal acquisitions, they are located centrally, anteriorly, and dorsally. On axial images, plaques are located in the lateral segments and have wedge shape with the basis at the cord surface or round shape if there is no contact with the cord surface [172].
Unlike SCI, MS predominantly involves cervical cord (62 %) and usually demonstrates relapsing-remitting disease course [172]. Simultaneous imaging of the brain to detect synchronous lesions helps to differentiate MS from cord infarction. Up to 92 % of MS patients with cord lesions have associated brain lesions [177] (Fig. 14). Detection of synchronous lesions in brain and spinal cord is useful in differentiating the MS from other inflammatory and cerebrovascular diseases with an accuracy of 93–95 % [178–180].
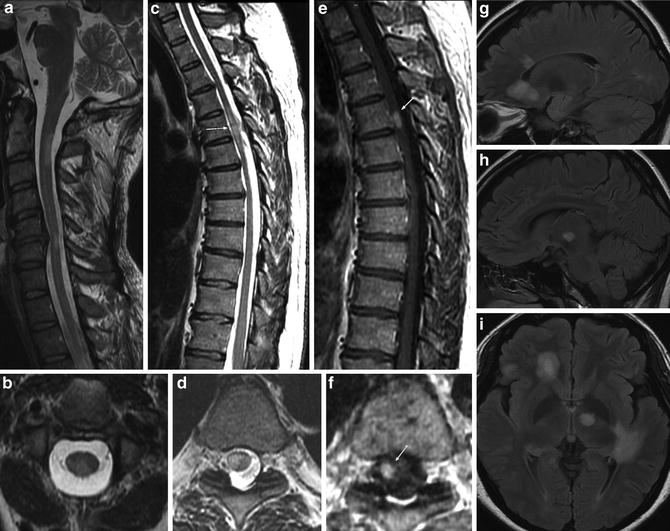
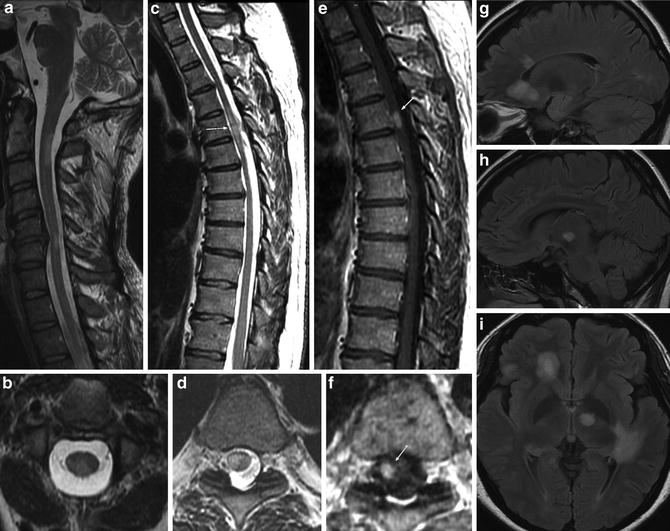
Fig. 14
Multiple sclerosis: a 41-year-old female presenting with relapsing-remitting course of neurological deficits. MRI – multiple T2-WI hyperintense demyelination plaques involving the cervical (a, b) and thoracic (c, d) spinal cord. MS plaques are peripherally located and occupy less than half the cross-sectional area of the cord (b, d, f). The thoracic cord lesion shows post-contrast enhancement (e, f) suggestive of active demyelination plaque. Simultaneous brain MRI shows multiple intracranial white matter lesions with distribution of multiple sclerosis involving the corpus callosum and peritrigonal distribution (g–i)
Idiopathic Transverse Myelitis (ITM)
Transverse myelitis (TM) is predominantly immune system mediated [181]. This may exist as an isolated idiopathic entity or as a part of the systemic process with reported incidence in patients with vasculitis, neurosarcoidosis, multiple sclerosis, and systemic lupus erythematosus [182] and following vaccination [183, 184] and acute gastrointestinal and respiratory illness [181].
ITM has reported a prevalence of 15.6 % in a retrospective study involving 288 patients with transverse myelitis [185]. ITM predominantly affects the middle-aged adults. The term “transverse” is self-explanatory describing the position of the inflammation, that is, across the width of the spinal cord. Thoracic cord is most commonly involved [182]. The criteria proposed by the Transverse Myelitis Consortium Working Group for idiopathic transverse myelitis [186] include:
1.
Bilateral sensory, motor, or autonomic spinal cord dysfunction
2.
Defined sensory level with bilateral signs and symptoms
3.
Proof of spinal cord inflammation on MRI or CSF
4.
Duration between symptoms’ onset to maximum intensity: few hours to 21 days
5.
Exclusion of extra-axial compressive etiology
Typical MR findings of ITM [182] include focal centrally located T2-WI hyperintensity, usually occupying more than two-thirds of the spinal cord cross-sectional area (88 %), signal abnormality that extends for more than three to four vertebral segments in length (53 %), variable presence of cord swelling or expansion (47 %), and patchy or diffuse post-contrast enhancement (47–53 %) [182, 185]. The variability in the duration of clinical onset, extent, and pattern of cord signal abnormality and given up to 40 % of ITM cases can have normal MR imaging [187] make it quite challenging to differentiate ITM from cord infarction (Fig. 15). A detailed history of associated systemic conditions, through clinical examination and close monitoring of the clinical condition in combination with CSF analysis, may be helpful in differentiating these two entities. A history of recent vaccination or viral illness may suggest ITM, but these are often absent. Symptoms isolated to the anterior spinal artery territory suggest infarction over inflammation, but this is not definitive. Progression of symptoms over at least a few hours, symptoms and MRI lesions that extend beyond a vascular territory, gadolinium enhancement on the initial presentation, and cerebrospinal fluid findings of pleocytosis or elevated IgG levels favor transverse myelitis over infarction [188].
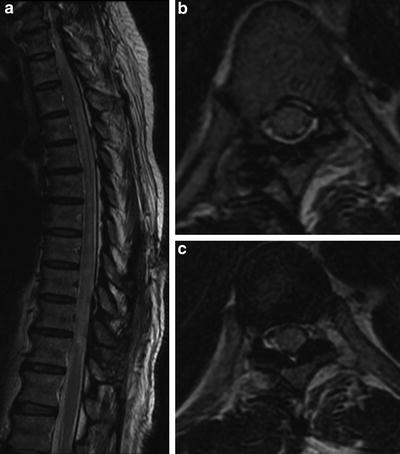
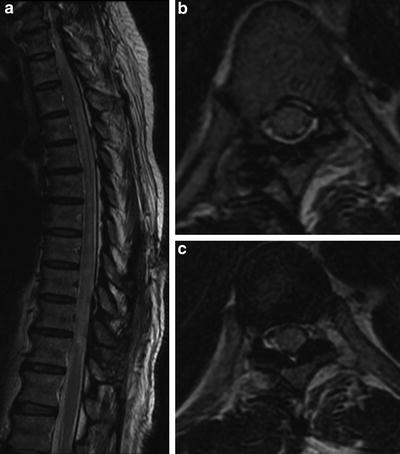
Fig. 15
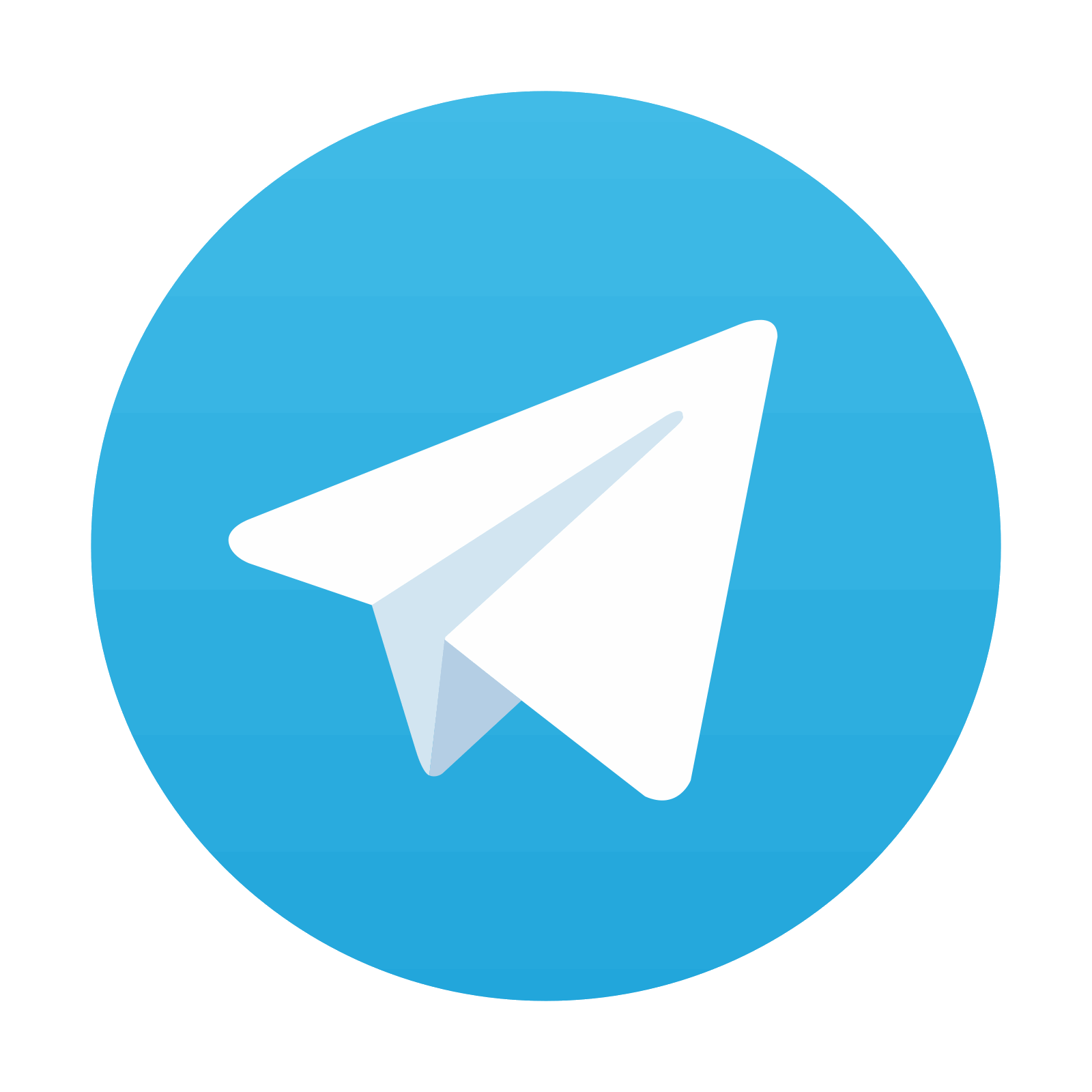
Idiopathic transverse myelitis: a 23-year-old female with abrupt onset paraplegia. Diffuse T2-WI hyperintense cord signal abnormality with edema and cord swelling (a–c). Note the signal abnormality does not follow a specific arterial territory and involves more than two-thirds of the cross-sectional area of the spinal cord (b, c). No associated intracranial lesions are demonstrated on the MRI brain performed simultaneously
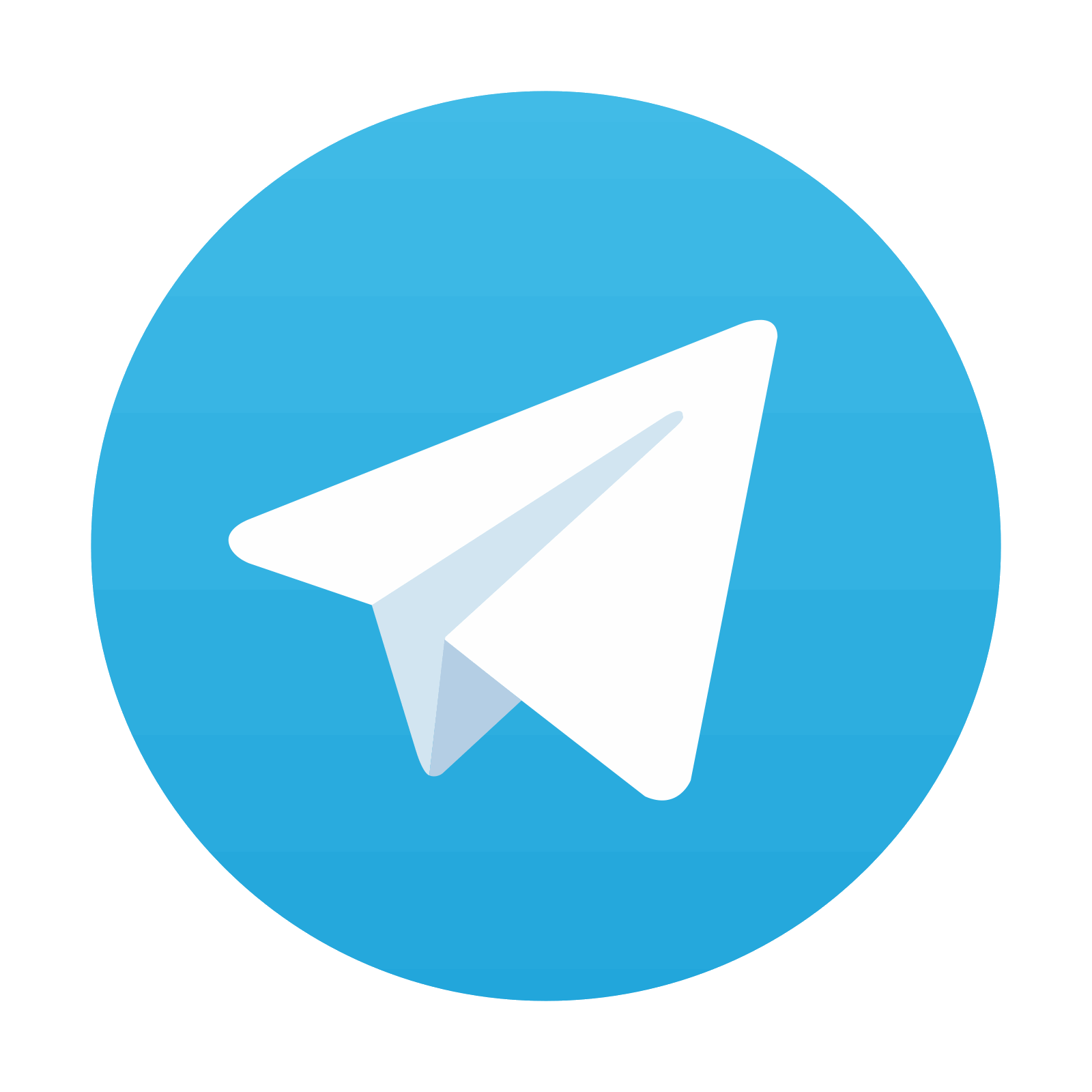
Stay updated, free articles. Join our Telegram channel
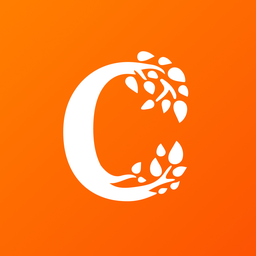
Full access? Get Clinical Tree
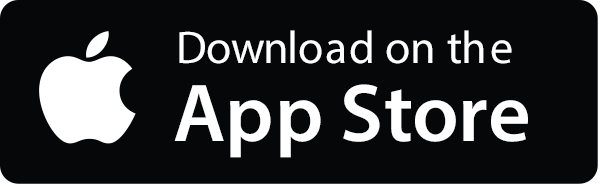
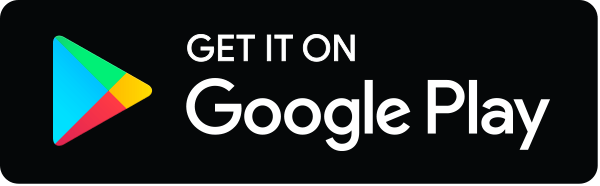
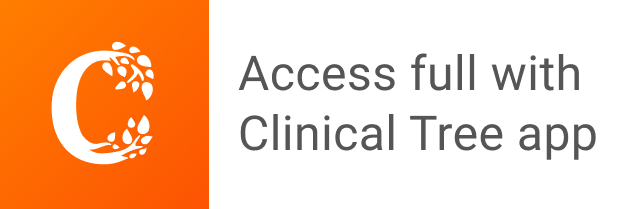