Fig. 9.1
a Areas of relative overactivity in normal controls compared with patients with idiopathic Parkinson disease (iPD) during a complex sequential right-hand movement (z = location of area of activation above commissural plane; threshold = p < 0.01). b Areas of relative overactivity in patients with iPD compared with normal controls during a complex sequential right-hand movement, superimposed onto a stereotaxically normalized MRI brain scan (z = location of area of activation above commissural plane; threshold = p < 0.001). (Modified from Sabatini et al. [3] with permission from Oxford University Press)
The increased activity in the pre-SMA is highly correlated with improved motor performance. The pre-SMA is suggested to be critical in planning and decision-making of movements and might play a primary role in the preparation of self-initiated movements [10–12]. The pre-SMA is also one of the main target regions receiving projections from the basal ganglia motor circuit [13]. These results indicate that the dysfunction of pre-SMA, due to a deficit of the nigrostriatal dopamine system, is an important reason contributing to the akinesia in iPD. The hyperactivity in the premotor cortex, parietal cortex, and cerebellum is likely compensatory. iPD patients need recruitment of other motor circuits to compensate for the functional deficits of the striatum in order to overcome their difficulty in performing movements [3, 6, 7, 14–16].
The motor deficits in iPD appear not only during motor execution, but also in the motor learning process. BOLD fMRI studies of motor learning commonly found that iPD patients have dysfunction in frontostriatal motor circuits, especially with decreased activity in the prefrontal cortex, but with compensatory hyperactivation in the parietal and premotor cortices and cerebellum [17–19]. The difficulty of iPD patients in motor learning is made more obvious when they try to shift learned movements to the automatic stage. It has been shown that iPD patients need greater activity in the cerebellum, premotor area, parietal cortex, precuneus, and prefrontal cortex compared with normal subjects while performing automatic movements. They require more brain activity to compensate for basal ganglia dysfunction in order to perform automatic movements [6].
Functional MRI has also been used to investigate the deficits of iPD patients in more complex coordination motor tasks. For example, iPD patients have difficulty in performing bimanual movements, especially antiphase movements [20]. Antiphase bimanual movements are when both hands perform the same movement with a phase shift of 180° between the two hands (i.e., one hand flexes while the other extends). A recent study found that the SMA was more activated during antiphase movement than inphase movement in controls, but not in iPD patients. In performing antiphase movements, iPD patients showed less activity in the basal ganglia and SMA, and had more activation in the M1, premotor cortex, and cerebellum compared with normal subjects. These findings suggest that dysfunction of the SMA and basal ganglia, abnormal interactions of brain networks, and disrupted attentional networks are important reasons contributing to the difficulty in performing bimanual antiphase movements. More brain activity and stronger connectivity are required in some brain regions to compensate for dysfunction of the supplementary motor area and basal ganglia in order to perform bimanual movements correctly [8].
Idiopathic PD patients also have more difficulty in performing two different tasks simultaneously (dual task). Wu and Hallett [21] found that iPD subjects can execute some simple dual motor and cognitive tasks correctly, but needed greater activity in the cerebellum, premotor area, parietal cortex, precuneus, and prefrontal cortex compared with controls. These fMRI findings suggest that the problem in performing dual tasks in iPD is due to limited attentional resources, defective central executive function, and less automaticity in performing the tasks.
More recently, fMRI has been used to investigate the alterations in connectivity of motor circuits. Since iPD affects large-scale brain networks, examination of network interactions may provide more valuable information in understanding pathophysiological changes than simply investigating local brain activity. The method used to explore network integration is analysis of functional or effective connectivity. Functional connectivity is defined as the degree of temporal correlation between spatially remote brain regions, whereby areas having more similar blood flow responses over time are considered to be more “functionally connected.” Effective connectivity expands on this by predicting the direction of information flow between two regions and is defined as the influence one neuronal system exerts over another [22]. These methods are increasingly being used to investigate iPD-induced modifications of brain networks [23–31]. The primary finding of these studies is that in addition to regional activation changes, the connectivity of brain networks also has iPD-related modifications during the performance of various motor tasks. For example, using structural equation modeling (SEM) [23], psychophysiological interaction (PPI) [30, 31], and dynamic causal modeling (DCM) [24], investigators have identified that iPD patients fail to modulate effective connectivity between prefrontal cortex, premotor cortex, and SMA. During the performance of self-initiated movement, the connectivity of striatal motor circuits is weakened, which might contribute to the impairment in performing self-initiated movements in iPD. Meanwhile, the connections between corticocerebellar motor regions are strengthened and may help compensate for basal ganglia dysfunction ([31], Fig. 9.2). While the use of fMRI activation maps to measure the amplitude of blood flow responses may provide valuable information about regional brain changes, connectivity analyses may be a more sensitive method to detect neural changes in iPD [32].
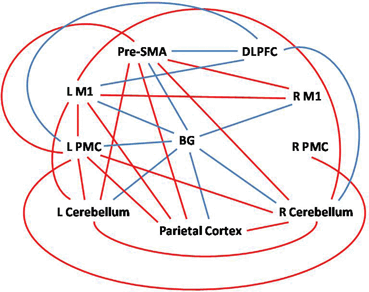
Fig. 9.2
Differences of effective connectivity between iPD patients and healthy controls among the basal ganglia, rostral supplementary motor area (pre-SMA), bilateral primary motor cortex (M1), bilateral premotor cortex (PMC), bilateral cerebellum, dorsolateral prefrontal cortex (DLPFC), and parietal cortex. Red/blue lines indicate increased/decreased connectivity between the two brain regions in iPD patients compared with healthy controls. (Modified from Wu et al. [31] with permission from Elsevier)
Functional MRI Studies of Nonmotor Symptoms of Idiopathic PD
Besides motor symptoms, most iPD patients present with nonmotor symptoms (e.g., cognitive, emotional, or olfactory impairments). These problems can predate the onset of motor symptoms, and may be due to degeneration outside the basal ganglia, although dysfunction of the basal ganglia also contributes to some nonmotor symptoms. The basal ganglia were classically regarded as motor structures. However, in recent years, it has been recognized that the basal ganglia have an important role in diverse functions, including cognitive and emotional control. Using fMRI and functional connectivity methods, Di Martino et al. [33] provided evidence for the hypothesized motor (dorsal putamen), cognitive (ventral putamen, dorsal caudate, superior ventral striatum), and affective (inferior ventral striatum) subdivisions of the striatum.
Cognitive deficits are very common in iPD. fMRI studies revealed that cognitively impaired PD patients had significantly less activation in the prefrontal cortex compared with unimpaired patients or healthy controls [34–36]. Some studies observed compensatory hyperactivation in the inferior frontal and posterolateral temporal-parietal areas in iPD patients [34]. Monchi et al. [37] have developed a card-sorting task to study the role of the caudate nucleus in executive processes, and shown that the caudate nucleus is specifically required when a set-shift must be planned. Using this task, the same group [38] observed increased cortical activation in iPD patients compared with the control group in the condition not specifically requiring the caudate nucleus, whereas decreased cortical activation was observed in the patients in the condition significantly involving the caudate nucleus. In addition, this study provided evidence that not only the nigrostriatal, but also the mesocortical dopaminergic pathway may play a significant role in the cognitive deficits in PD. van Eimeren et al. [28] examined the integrity of the so-called “default mode network” (DMN). The DMN is a network showing consistent task-related deactivations, and includes the medial prefrontal cortex, anterior cingulate cortex, posterior cingulate cortex, precuneus, and inferior parietal lobe [39, 40]. The functional role of this network is thought to facilitate cognitive performance by allocating neural resources to critical brain regions. iPD patients showed less deactivation of the posterior cingulate cortex and the precuneus compared with controls during performing a card-sorting task, while medial prefrontal cortex and the rostral ventromedial caudate nucleus were functionally disconnected. The malfunctioning of the DMN may contribute to the executive deficits in iPD.
Impulse control disorders, including gambling or compulsive shopping, are frequently observed in iPD patients treated with dopamine agonists [41]. A couple of recent fMRI studies [42, 43] found that during performance of a probabilistic learning task in which participants had to learn whether a symbol was associated with reward or loss [42], iPD patients with impulse control disorders showed an increased striatal prediction error signal in the gain condition after intake of dopamine agonists. This enhanced response in ventral striatum signaled a better than expected outcome for chosen actions and suggesting this might bias behavioral decisions in these patients. When performing a risk-taking task, iPD patients with impulse control disorders made more risky choices in the gain condition along with decreased orbitofrontal cortex and anterior cingulate activations [43]. The opposite pattern was found in iPD patients without these symptoms. Dopamine agonists have also been shown to decrease activation in the ventral striatum and biased decisions toward greater risk in iPD patients with impulse control disorders.
Hallucinations are also present in some iPD patients. Patients with visual hallucinations showed greater activation in the inferior frontal gyrus and the caudate nucleus, and less activation in the parietal lobe and cingulate gyrus compared with patients without hallucinations during stroboscopic (flashing) visual stimulation. During kinematic (apparent motion) stimulation, iPD subjects with hallucinations showed greater activation in the superior frontal gyrus, and less activation in area V5/MT, parietal cortices, and cingulate gyrus compared with iPD subjects with no hallucinations. These results indicate iPD patients with chronic visual hallucinations respond to visual stimuli with greater frontal and subcortical activation and less visual cortical activation than those without hallucinations. Shifting visual circuitry from posterior to anterior regions associated primarily with attention processes suggests altered network organization may play a role in the pathophysiology of visual hallucinations in iPD [44].
Depression is the most frequent psychiatric disorder in iPD. Using an event-related parametric emotional perception paradigm, Cardoso et al. [45] showed decreased activation in the left mediodorsal thalamus and medial prefrontal cortex in iPD patients with depression compared with those without depression. These findings highlight the importance of limbic thalamus in iPD depression.
Olfactory impairment can be a premotor symptom in iPD. An event-related fMRI during an odor-detection task found olfactory-induced hyperactivation in piriform and orbitofrontal cortices in iPD patients [46]. The authors defined trials without olfactory stimulation as noise trials and trials with olfactory stimulation as signal trials, referring to the fact that additional information, namely the signal, is superimposed upon the noise. They found that while orbitofrontal areas seem to be unable to discriminate between signal and noise, primary olfactory cortex shows preserved discriminatory ability. These results support a complex network dysfunction that exceeds structural pathology observed in the olfactory bulb and mesolimbic cortices.
Use of Resting-State Functional MRI in Idiopathic PD
In recent years, the use of so-called resting-state fMRI (RS-fMRI) methodology has been developed to measure neural functional connectivity based on the presence of spontaneous low-frequency fluctuations of BOLD signal [47]. Compared with the conventional task-based fMRI method, RS-fMRI can circumvent task-related confounds, increase the signal-to-noise ratio, and expand patient populations [48]. RS-fMRI has been used to investigate iPD-related spontaneous neural activity. Wu et al. [49] used regional homogeneity (ReHo), a method to analyze the BOLD signal of the brain to examine changes in the iPD brain during rest. ReHo [50] assumes that within a functional cluster, the hemodynamic characteristics of every voxel would be similar or synchronous with that of each other, and such similarity could be changed or modulated by different conditions. The authors observed decreased ReHo in the putamen, thalamus, SMA, and increased ReHo in the cerebellum, M1, and premotor area in iPD patients compared with healthy controls (Fig. 9.3). The ReHo in the off-levodopa condition was negatively correlated with the Unified Parkinson’s Disease Rating Scale (UPDRS) in the putamen, and was positively correlated with the UPDRS in the cerebellum. Administration of levodopa relatively normalized ReHo. These findings demonstrate that there are iPD-related specific changes of neural activity in the resting state . Moreover, some of these changes are secondary to dopamine deficiency, and relate to the severity of the disease.
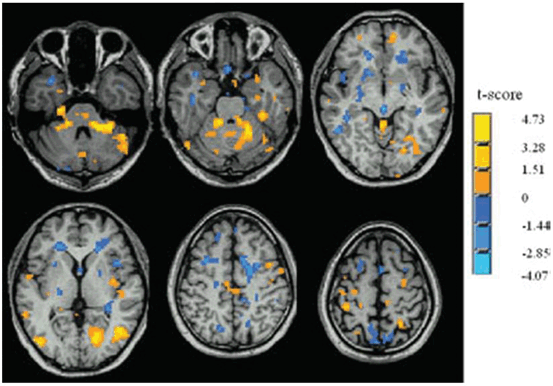
Fig. 9.3
Idiopathic Parkinson disease-related changes of regional homogeneity (ReHo) shown as a comparison of Kendall’s coefficient of concordance (KCC) maps between patients with iPD in the off state and controls (two-sample t test; p < 0.05, corrected) in the resting state. t-score bars are shown on the right. Hot and cold colors indicate iPD-related ReHo increases and decreases, respectively. (Modified from Wu et al. [49] with permission from John Wiley and Sons)
The amplitudes of the spontaneous low-frequency fluctuations (ALFF) have also been explored in iPD [51]. Findings include decreased amplitudes of BOLD in the SMA, mesial prefrontal cortex, right middle frontal gyrus, left cerebellum, and increased amplitudes in the right cerebellum. These patterns were able to distinguish between iPD patients and healthy controls with 92 % sensitivity and 87 % specificity [52]. These findings suggest that RS-fMRI has the potential to be developed as a biomarker of iPD.
Resting-state fMRI has also been used to evaluate activity patterns associated with the presence of apathy or depression in iPD [53]. The authors found that the apathy score was best predicted by ALFF signal in the left supplementary motor cortex, the right orbitofrontal cortex, and the right middle frontal cortex, whereas depression score is best predicted by ALFF signal in the right subgenual cingulate. Disease severity was best predicted by ALFF signal in the right putamen.
Resting-state fMRI has been proved well suited for network connectivity studies in iPD. A RS-fMRI study with a network model based on graph theory compared the functional connectivity in the motor network between iPD patients and healthy controls [54]. Patients off medication had decreased connectivity in the SMA, left dorsolateral prefrontal cortex, and left putamen, and increased connectivity in the left cerebellum, left M1, and left parietal cortex. Administration of levodopa relatively normalized these connectivity patterns in patients. Using multiple regression analyses, Helmich et al. [55] investigated striatal connectivity in iPD patients. They found that the posterior putamen had reduced coupling with the inferior parietal cortex, but had increased connectivity with the anterior putamen in iPD. They concluded that dopamine depletion lead to a remapping of cerebral connectivity that reduced the spatial segregation between different corticostriatal loops, which might contribute to the abnormal sensorimotor integration in iPD. RS-fMRI has also been used to identify iPD-related changes in the connectivity profile of cortical motor areas [56] and the subthalamic nucleus [57]. A recent RS-fMRI study found that iPD with dementia is associated with selective disruption of corticostriatal connectivity [58].
Functional MRI in Preclinical Idiopathic PD
The neurodegenerative process in iPD begins years before the onset of any clinical symptoms [59] and the development of dopaminergic degeneration [60]. As a reliable method to identify individuals at risk for developing sporadic PD is not yet available, an alternative approach is to use asymptomatic genetic mutation carriers to study pathophysiological changes of preclinical iPD. fMRI studies have reported neural changes in some types of asymptomatic mutation carriers such as Parkin and PINK1 [61–63]. These studies have found additional recruitment of SMA, cingulate motor area, and premotor cortex in asymptomatic mutation carriers compared with healthy controls during motor tasks. In addition, the cingulate motor area had a stronger influence on activity in the basal ganglia in asymptomatic mutation carriers compared with controls (Fig. 9.4). These results might reflect a compensatory reorganization of striatocortical motor loops to maintain motor function in the preclinical stage.
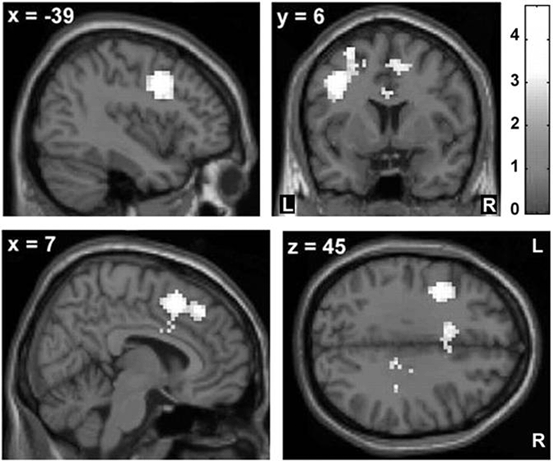
Fig. 9.4
The z-score maps delineate voxels with relative overactivity during internally selected movements in asymptomatic carriers of a single mutant Parkin allele relative to healthy noncarriers (p < 0.05, corrected at the cluster level). Asymptomatic carriers showed increased activity in the rostral cingulate motor area (rCMA) and adjacent rostral supplementary motor area (SMA) as well as the left dorsal premotor cortex (PMd). (Modified from Buhmann et al. [61] with permission from Oxford University Press)
Use of Perfusion MRI in Idiopathic PD
Arterial spin labeling (ASL) is a noninvasive MRI perfusion method that quantitatively measures CBF per unit of tissue mass [64]. ASL eliminates expensive, potentially harmful radioactive materials and long preparation times and requires shorter scan times than radiotracer methods. It is an easily repeatable addition to routine MRI scanning that produces absolute perfusion images. Melzer et al. [65] used pseudo-continuous ASL and principal component analysis to derive an iPD-related perfusion network via logistic regression. They found an iPD-related pattern of decreased perfusion in the posterior parieto-occipital cortex, precuneus and cuneus, and middle frontal gyri compared with healthy controls. Perfusion was preserved in globus pallidus, putamen, anterior cingulate, and postcentral and precentral gyri. Both motor and cognitive statuses were significant factors related to network score. Another study compared the iPD-related spatial covariance pattern (PDRP) derived from continuous ASL perfusion MRI with the corresponding 18F-fluorodeoxyglucose PET (FDG-PET) values. The PDRP expression was abnormally elevated in patients scanned with either modality, and the values from ASL-MRI and FDG-PET were highly intercorrelated (p < 0.0001). Perfusion MRI methods can be used for accurate quantification of disease-related covariance patterns [66]. These results suggest that perfusion MRI has potential to be a functional biomarker of PD.
Functional MRI in Atypical Parkinsonism
Functional MRI has also been used to investigate neural changes in atypical parkinsonian disorders (aPD) such as multiple system atrophy (MSA) , progressive supranuclear palsy (PSP), and dementia with Lewy bodies (DLB). These studies have found disorder-specific activity or connectivity patterns that may help to distinguish iPD from aPD. You et al. [67] found that MSA patients had decreased ReHo in the left M1, posterior cingulate cortex, left lateral prefrontal cortex, and right inferior parietal lobule, and had increased ReHo in the right M1, bilateral premotor cortices, bilateral SMA, medial prefrontal cortex, and left inferior parietal lobule.
Whitwell et al. [68] found reduced functional connectivity between the thalamus and premotor cortex, and the SMA, striatum, and cerebellum. Reduced connectivity in the basal ganglia network, DMN, and subcortical salience networks were also present in patients with PSP. SMA functional connectivity correlated with SMA volume and measures of cognitive and motor dysfunction, while thalamic connectivity correlated with degeneration of superior cerebellar peduncles. These results suggest that PSP is associated with disrupted thalamocortical connectivity that is associated with degeneration of the dentatorubrothalamic tracts and the presence of cortical atrophy. Zwergal et al. [69] used mental imagery of standing during fMRI , and revealed a reduced activation of the mesencephalic brainstem tegmentum and the thalamus in PSP patients with postural instability and falls. The results give support to the hypothesis that reduced thalamic activation via the ascending brainstem projections may cause postural imbalance in PSP.
< div class='tao-gold-member'>
Only gold members can continue reading. Log In or Register a > to continue
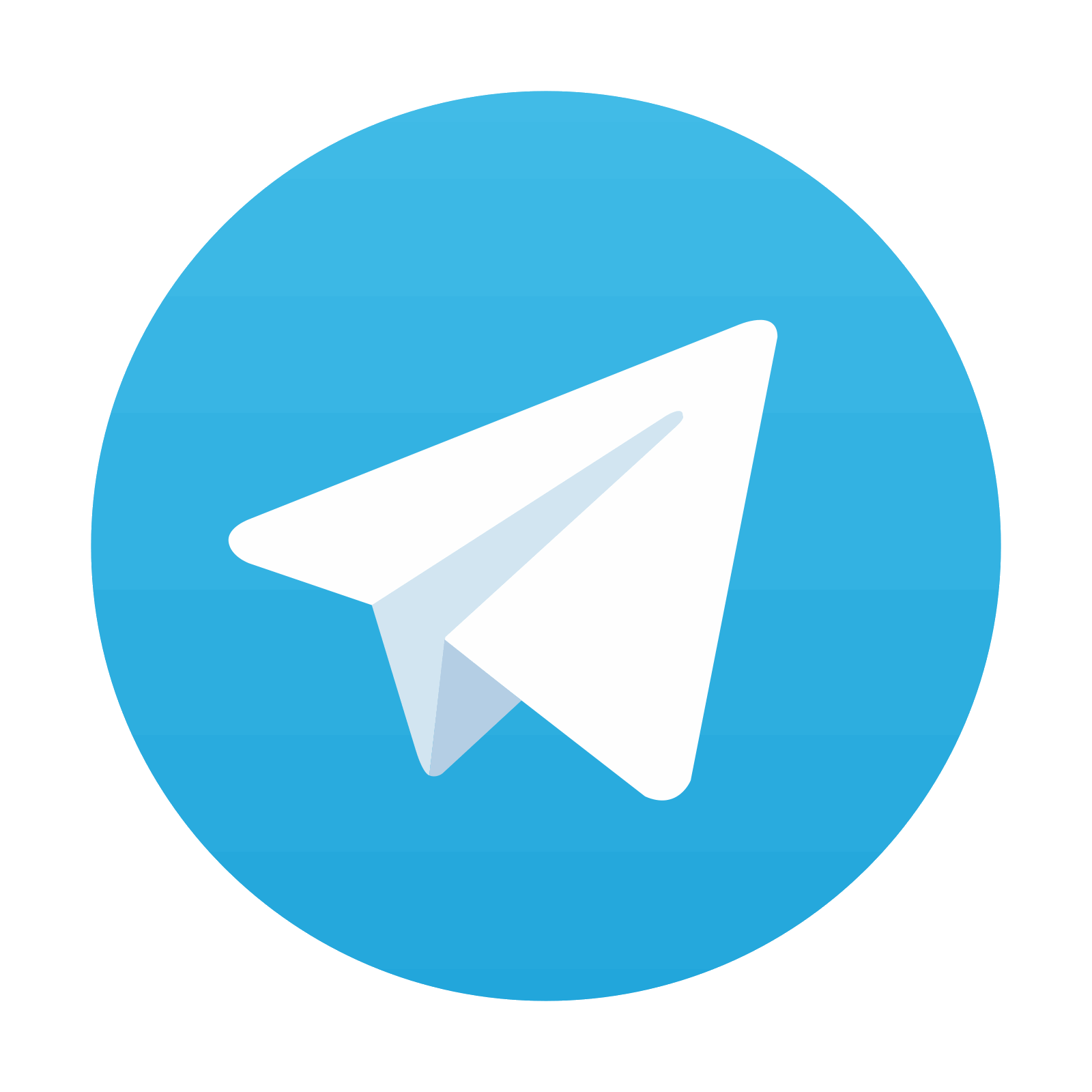
Stay updated, free articles. Join our Telegram channel
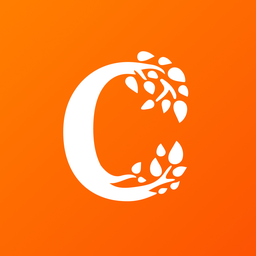
Full access? Get Clinical Tree
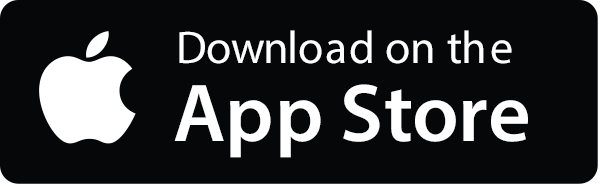
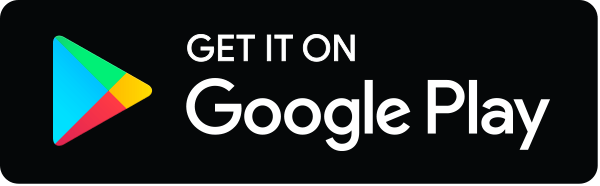